Forms of Energy

Energy is defined as the ability to do work. Energy comes in various forms. Here are 10 common types of energy and examples of them.
Mechanical energy
Mechanical energy is energy that results from movement or the location of an object. Mechanical energy is the sum of kinetic energy and potential energy.Examples: An object possessing mechanical energy has both kinetic and potential energy, although the energy of one of the forms may be equal to zero.
A moving car has kinetic energy. If you move the car up a mountain, it has kinetic and potential energy. A book sitting on a table has potential energy.
Thermal energy
Thermal energy or heat energy reflects the temperature difference between two systems.Example: A cup of hot coffee has thermal energy. You generate heat and have thermal energy with respect to your environment.
Nuclear energy
Nuclear energy is energy resulting from changes in the atomic nuclei or from nuclear reactions.Example: Nuclear fission, nuclear fusion, and nuclear decay are examples of nuclear energy. An atomic detonation or power from a nuclear plant are specific examples of this type of energy.
Chemical energy
Chemical energy results from chemical reactions between atoms or molecules. There are different types of chemical energy, such as electrochemical energy and chemiluminescence.Example: A good example of chemical energy is an electrochemical cell or battery.
Electromagnetic energy
Electromagnetic energy (or radiant energy) is energy from light or electromagnetic waves.Example: Any form of light has electromagnetic energy, including parts of the spectrum we can't see. Radio, gamma rays, x-rays, microwaves, and ultraviolet light are some examples of electromagnetic energy.
Sonic energy
Sonic energy is the energy of sound waves. Sound waves travel through air or another medium.Example: A sonic boom, a song played on a stereo, your voice
Gravitational energy
Energy associated with gravity involves the attraction between two objects based on their mass. It can serve as a basis for mechanical energy, such as the potential energy of an object placed on a shelf or the kinetic energy of the Moon in orbit around the Earth.Example: Gravitational energy holds the atmosphere to the Earth.
Kinetic energy
Kinetic energy is the energy of motion of a body. It ranges from 0 to a positive value.Example: An example is a child swinging on a swing. No matter whether the swing is moving forward or backward, the value of the kinetic energy is never negative.
Potential energy
Potential energy is the energy of an object's position.Example: When a child swinging on a swing reaches the top of the arc, she has maximum potential energy. When she is closest to the ground, her potential energy is at its minimum (0). Another example is throwing a ball into the air. At the highest point, the potential energy is greatest. As the ball rises or falls it has a combination of potential and kinetic energy.
Ionization energy
Ionization energy is the form of energy that binds electrons to the nucleus of its atom, ion, or molecule.Example: The first ionization energy of an atom is the energy needed to remove one electron completely. The second ionization energy is energy to remove a second electron and is greater than that required to remove the first electron.
In a Flash
Forms of Energy
While energy can come in many different types, there are only two main forms of energy: kinetic energy and potential energy. These energies are found in all objects.If an object is in motion (i.e. moving), it is said to have kinetic energy.
Energy stored within an object – due to the object's position, arrangement or state - is known as potential energy.
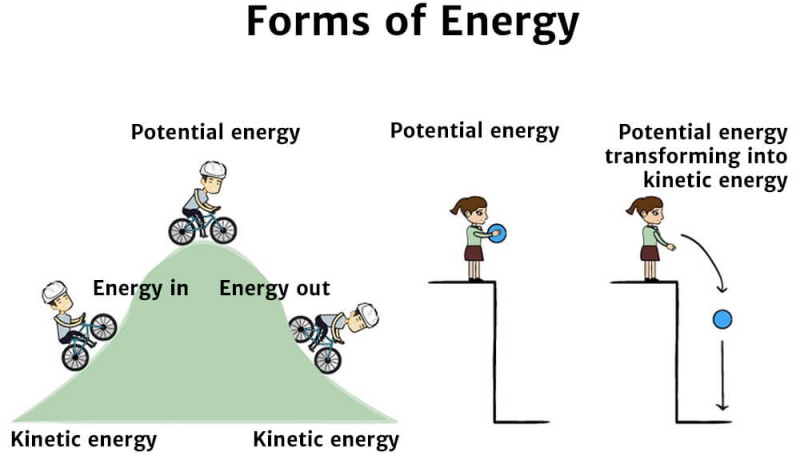
Objects in motion have kinetic energy; stationary objects can hold potential energy.

What is kinetic energy?
Kinetic energy is the energy of motion. If an object is moving, it has kinetic energy.The kinetic energy of an object is calculated based on two factors:
- Velocity: The speed the object is moving in a particular direction
- Mass: How much matter is in the object (this is usually measured by the weight of the object)
What are some examples of kinetic energy?
Examples of kinetic energy include:- An airplane in flight
- A ball being thrown
- A car travelling on a road
- A river flowing
- A person running

What is potential energy?
Potential energy is energy that is stored in an object or substance. This stored energy is due to the position, arrangement or state of the object or substance.You can think of it as energy that has the 'potential' to do work. When the position, arrangement or state of the object changes, the stored energy will be released.
What are some examples of potential energy?
Examples of potential energy include:- A rock sitting on the edge of a cliff. If the rock falls, the potential energy will be converted to kinetic energy, as the rock will be moving.
- A stretched elastic band in a slingshot. When the elastic band is released, it will cause its projectile to shoot forward.
- A stick of dynamite. The chemical potential energy in dynamite will be released when the fuse is lit and comes into contact with the chemicals.
- The food we eat. Food contains chemical potential energy – as our bodies digest it, the stored energy is converted into energy for us to move and grow.
What Do You Mean?
Kinetic energy is the energy of a moving object.Potential energy is energy that is stored in an object or substance.
Velocity is the speed of a moving object in a particular direction.
Mass is the amount of matter container within an object.
Speedy Summary

Why did the firm hire the man standing on the edge of a hill?
They thought he had potential!XXX . XXX Forms of Energy and Energy Transformations
Introduction
Energy is the ability to do work. It is one of the basic human needs and is an essential component in any development programme. In this lesson, we are going to look at the forms that energy exists, namely: heat, light, sound, electrical, chemical, nuclear and mechanical. These forms of energy may be transformed from one form to the other, usually with losses.
Objectives
By the end of this lesson you should be able to:
|
Heat
Heat energy, also referred to as thermal energy, is really the effect of moving molecules. Matter is made up of molecules, which are in continual motion and in a solid, vibrate about a mean position. The motion of any molecule increases when the energy of the substance is increased. This may cause an increase in the temperature of the substance or lead to a change of state. The higher the temperature, the greater the internal energy of the substance.
Heat energy is the most easily lost or dissipated form of energy. It is also the form of energy into which other forms of energy can easily change. However, heat can be changed into other forms of energy with a lot of waste.also called Solar Energy
Light
Light energy is a type of wave motion. That is, light is a form of energy caused by light waves. It enables us to see, as objects are only visible when they reflect light into our eyes.Sound
Sound energy is also a type of wave motion. We are heard by others when we talk because of the sound energy we produce. It is due to the effect of the air molecules vibrating when we talk. The vibrating molecules hit our eardrums, which enable us to hear others talk. Sound energy may be converted into electrical energy for transmission, and later the electrical energy can be converted back into sound energy at the receiving end. An example of such transformations could be seen in the microphone and the loudspeaker.Sound, like heat energy is easily lost. The transformation of one form of energy into another may be accompanied by losses in the form of sound and/or heat that are often not desirable.
Electrical Energy
Electrical energy is really the effect of moving electrical charges from one point to another in a conductor. Electrical charges moving through a conductor is called electricity. Electrical energy may be easily changed into other forms of energy to suit our particular needs. Lightning is an example of electrical energy. Electric current is the means by which electrical energy is most easily transported to places where it is needed and converted into other forms.Chemical Energy
This is the energy stored within chemical compounds. A chemical compound is formed by the rearrangement of atoms that is accompanied by energy loss or gain. This energy is the chemical energy gained or lost in the formation of the compound.Food, biomass, fuel and explosives have a store of chemical energy. The energy from food is released by chemical reactions in our bodies in the form of heat. Fuels like coal, oil and natural gas contain chemical energy that may be converted into other forms of energy like heat and light. The chemical energy present in a given fuel is determined by its calorific value – the heat liberated when 1 Kg of the fuel is burnt. Batteries and explosives also contain chemical energy that could be converted into other forms of energy, some beneficial, others harmful.
Nuclear Energy
Nuclear Energy, also known as atomic energy, is energy stored in the nucleus of an atom. It is this energy that holds the nucleus together and could be released when the nuclei are combined (fusion) or split (fission) apart. Nuclear energy can be used for peaceful purpose as well as destructive purposes (as in the atomic bomb). Considering peaceful purposes, nuclear energy is used to generate electricity in nuclear power plants, produce steam for driving machines, powering some submarines and spacecrafts. In these applications, the nuclei of uranium atoms are split in a process called fission. Nuclear energy is also the source of the sun’s energy. The sun combines the nuclei of hydrogen atoms into helium atoms in a process called fusion.Albert Einstein put forward the following equation that provides the basis for calculating the amount of energy released when the nucleus of an atom is split. E = mc2
The equation means that when the nucleus of an atom is split, the amount of energy released, E, in joules, is equal to the loss of its mass, m, in kilograms, times the speed of light squared, c2, in m/s2. Because c2 is a very large figure (300,000,000 X 300,000,000 = 90,000.000,000,000,000 ), a small amount of mass can be converted into an enormous amount of energy. An atom of uranium splits into two smaller atoms and loses roughly 0.1 percent of its mass that is converted into a vast amount of energy. One-kilogram mass of any substance completely converted into energy equals 90,000,000,000,000,000 J or 25 billion kilowatt hours!! This is more than the electrical energy needs of the United States for two days!!
Mechanical Energy
Mechanical energy is the kind of energy that can do mechanical work directly. Naturally occurring sources of mechanical energy include winds, waterfalls and tides.There are two kinds of mechanical energy, namely kinetic energy and potential energy. Kinetic energy is the energy a body possesses by virtue of its motion. A moving body of mass, m Kg and velocity v m/s possesses kinetic energy of magnitude
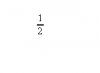
Potential energy is the energy of a body due to its position or shape. This form of energy could be considered as energy stored in a body to be released when it begins to move or change its position or shape. Quite often, potential energy changes to kinetic energy. A ball at the top of a slope, water behind a dam, a compressed spring and a stretched elastic band possess potential energy. For a body of mass, m Kg located at a height h metres above a particular chosen level such as ground level or sea level, its potential energy is mgh joules, where g = acceleration due to gravity, in m/s2. For an elastic spring stretched x m, its potential energy is (1/2)kx2 joules, where k = spring constant, in N/m.
Energy Conversion
One important property of energy is its ability to change from one form to another form. For example, chemical energy from fossil fuels (coal, oil and natural gas) can be converted into heat energy when burned. The heat energy may be converted into kinetic energy in a gas turbine and finally into electrical energy by a generator. The electric energy may subsequently be converted into light, sound or kinetic energy in our homes through various household appliances.During any energy conversion, the amount of energy input is the same as the energy output. This concept is known as the law of conservation of energy and sometimes referred to as the First Law of Thermodynamics. This law states: energy cannot be created nor destroyed but can be transformed from one form to another. Thus, the total energy of an isolated system is always constant and when energy of one form is expended an equal amount of energy in another form is produced. In every energy conversion, some high-grade energy is converted into low-grade energy as heat. Thus, the total amount of low-grade energy in the universe is increasing while high-grade energy is decreasing. Even though energy is never destroyed, we usually complain that the world is suffering from an energy shortage. Indeed we are suffering from shortage of high-grade energy that has the potential of producing useful power! Energy may change form, but the total amount of energy in the universe stays the same.
Reflection
Let us consider the following energy transformations that we encounter on daily basis in our everyday activities.
|
XXX . XXX 4%zero Energy
In physics, energy is the quantitative property that must be transferred to an object in order to perform work on, or to heat, the object.[note 1] Energy is a conserved quantity; the law of conservation of energy states that energy can be converted in form, but not created or destroyed. The SI unit of energy is the joule, which is the energy transferred to an object by the work of moving it a distance of 1 metre against a force of 1 newton.
Common forms of energy include the kinetic energy of a moving object, the potential energy stored by an object's position in a force field (gravitational, electric or magnetic), the elastic energy stored by stretching solid objects, the chemical energy released when a fuel burns, the radiant energy carried by light, and the thermal energy due to an object's temperature.
Mass and energy are closely related. Due to mass–energy equivalence, any object that has mass when stationary (called rest mass) also has an equivalent amount of energy whose form is called rest energy (in that frame of reference), and any additional energy (of any form) acquired by the object above that rest energy will increase the object's total mass just as it increases its total energy. For example, after heating an object, its increase in energy could be measured as an increase in mass, with a sensitive enough scale.
Living organisms require available energy to stay alive, such as the energy humans get from food. Human civilization requires energy to function, which it gets from energy resources such as fossil fuels, nuclear fuel, or renewable energy. The processes of Earth's climate and ecosystem are driven by the radiant energy Earth receives from the sun and the geothermal energy contained within the earth.

The Sun is the source of energy for most of life on Earth. As a star, the Sun is heated to high temperatures by the conversion of nuclear binding energy due to the fusion of hydrogen in its core. This energy is ultimately transferred (released) out into space mainly in the form of radiant (light) energy.

In a typical lightning strike, 500 megajoules of electric potential energy is converted into the same amount of energy in other forms, mostly light energy, sound energy and thermal energy.

Thermal energy is energy of microscopic constituents of matter, which may include both kinetic and potential energy.
While these two categories are sufficient to describe all forms of energy, it is often convenient refer to particular combinations of potential and kinetic energy as its own form. For example, macroscopic mechanical energy is the sum of translational and rotational kinetic and potential energy in a system neglects the kinetic energy due to temperature, and nuclear energy which combines utilize potentials from the nuclear force and the weak force), among others.
Type of energy | Description |
---|---|
Mechanical | the sum of macroscopic translational and rotational kinetic and potential energies |
Electric | potential energy due to or stored in electric fields |
Magnetic | potential energy due to or stored in magnetic fields |
Gravitational | potential energy due to or stored in gravitational fields |
Chemical | potential energy due to chemical bonds |
Ionization | potential energy that binds an electron to its atom or molecule |
Nuclear | potential energy that binds nucleons to form the atomic nucleus (and nuclear reactions) |
Chromodynamic | potential energy that binds quarks to form hadrons |
Elastic | potential energy due to the deformation of a material (or its container) exhibiting a restorative force |
Mechanical wave | kinetic and potential energy in an elastic material due to a propagated deformational wave |
Sound wave | kinetic and potential energy in a fluid due to a sound propagated wave (a particular form of mechanical wave) |
Radiant | potential energy stored in the fields of propagated by electromagnetic radiation, including light |
Rest | potential energy due to an object's rest mass |
Thermal | kinetic energy of the microscopic motion of particles, a form of disordered equivalent of mechanical energy |
In 1843, James Prescott Joule independently discovered the mechanical equivalent in a series of experiments. The most famous of them used the "Joule apparatus": a descending weight, attached to a string, caused rotation of a paddle immersed in water, practically insulated from heat transfer. It showed that the gravitational potential energy lost by the weight in descending was equal to the internal energy gained by the water through friction with the paddle.
In the International System of Units (SI), the unit of energy is the joule, named after James Prescott Joule. It is a derived unit. It is equal to the energy expended (or work done) in applying a force of one newton through a distance of one metre. However energy is also expressed in many other units not part of the SI, such as ergs, calories, British Thermal Units, kilowatt-hours and kilocalories, which require a conversion factor when expressed in SI units.
The SI unit of energy rate (energy per unit time) is the watt, which is a joule per second. Thus, one joule is one watt-second, and 3600 joules equal one watt-hour. The CGS energy unit is the erg and the imperial and US customary unit is the foot pound. Other energy units such as the electronvolt, food calorie or thermodynamic kcal (based on the temperature change of water in a heating process), and BTU are used in specific areas of science and commerce.

Joule's apparatus for measuring the mechanical equivalent of heat. A descending weight attached to a string causes a paddle immersed in water to rotate.
Classical mechanics
In classical mechanics, energy is a conceptually and mathematically useful property, as it is a conserved quantity. Several formulations of mechanics have been developed using energy as a core concept.Work, a function of energy, is force times distance.
The total energy of a system is sometimes called the Hamiltonian, after William Rowan Hamilton. The classical equations of motion can be written in terms of the Hamiltonian, even for highly complex or abstract systems. These classical equations have remarkably direct analogs in nonrelativistic quantum mechanics.[4]
Another energy-related concept is called the Lagrangian, after Joseph-Louis Lagrange. This formalism is as fundamental as the Hamiltonian, and both can be used to derive the equations of motion or be derived from them. It was invented in the context of classical mechanics, but is generally useful in modern physics. The Lagrangian is defined as the kinetic energy minus the potential energy. Usually, the Lagrange formalism is mathematically more convenient than the Hamiltonian for non-conservative systems (such as systems with friction).
Noether's theorem (1918) states that any differentiable symmetry of the action of a physical system has a corresponding conservation law. Noether's theorem has become a fundamental tool of modern theoretical physics and the calculus of variations. A generalisation of the seminal formulations on constants of motion in Lagrangian and Hamiltonian mechanics (1788 and 1833, respectively), it does not apply to systems that cannot be modeled with a Lagrangian; for example, dissipative systems with continuous symmetries need not have a corresponding conservation law.
Chemistry
In the context of chemistry, energy is an attribute of a substance as a consequence of its atomic, molecular or aggregate structure. Since a chemical transformation is accompanied by a change in one or more of these kinds of structure, it is invariably accompanied by an increase or decrease of energy of the substances involved. Some energy is transferred between the surroundings and the reactants of the reaction in the form of heat or light; thus the products of a reaction may have more or less energy than the reactants. A reaction is said to be exergonic if the final state is lower on the energy scale than the initial state; in the case of endergonic reactions the situation is the reverse. Chemical reactions are invariably not possible unless the reactants surmount an energy barrier known as the activation energy. The speed of a chemical reaction (at given temperature T) is related to the activation energy E, by the Boltzmann's population factor e−E/kT – that is the probability of molecule to have energy greater than or equal to E at the given temperature T. This exponential dependence of a reaction rate on temperature is known as the Arrhenius equation.The activation energy necessary for a chemical reaction can be in the form of thermal energy.Biology

Basic overview of energy and human life.
Sunlight's radiant energy is also captured by plants as chemical potential energy in photosynthesis, when carbon dioxide and water (two low-energy compounds) are converted into the high-energy compounds carbohydrates, lipids, and proteins. Plants also release oxygen during photosynthesis, which is utilized by living organisms as an electron acceptor, to release the energy of carbohydrates, lipids, and proteins. Release of the energy stored during photosynthesis as heat or light may be triggered suddenly by a spark, in a forest fire, or it may be made available more slowly for animal or human metabolism, when these molecules are ingested, and catabolism is triggered by enzyme action.
Any living organism relies on an external source of energy—radiant energy from the Sun in the case of green plants, chemical energy in some form in the case of animals—to be able to grow and reproduce. The daily 1500–2000 Calories (6–8 MJ) recommended for a human adult are taken as a combination of oxygen and food molecules, the latter mostly carbohydrates and fats, of which glucose (C6H12O6) and stearin (C57H110O6) are convenient examples. The food molecules are oxidised to carbon dioxide and water in the mitochondria
-
- C57H110O6 + 81.5O2 → 57CO2 + 55H2O
-
- ADP + HPO42− → ATP + H2O
- gain in kinetic energy of a sprinter during a 100 m race: 4 kJ
- gain in gravitational potential energy of a 150 kg weight lifted through 2 metres: 3 kJ
- Daily food intake of a normal adult: 6–8 MJ
Earth sciences
In geology, continental drift, mountain ranges, volcanoes, and earthquakes are phenomena that can be explained in terms of energy transformations in the Earth's interior,[9] while meteorological phenomena like wind, rain, hail, snow, lightning, tornadoes and hurricanes are all a result of energy transformations brought about by solar energy on the atmosphere of the planet Earth.Sunlight may be stored as gravitational potential energy after it strikes the Earth, as (for example) water evaporates from oceans and is deposited upon mountains (where, after being released at a hydroelectric dam, it can be used to drive turbines or generators to produce electricity). Sunlight also drives many weather phenomena, save those generated by volcanic events. An example of a solar-mediated weather event is a hurricane, which occurs when large unstable areas of warm ocean, heated over months, give up some of their thermal energy suddenly to power a few days of violent air movement.
In a slower process, radioactive decay of atoms in the core of the Earth releases heat. This thermal energy drives plate tectonics and may lift mountains, via orogenesis. This slow lifting represents a kind of gravitational potential energy storage of the thermal energy, which may be later released to active kinetic energy in landslides, after a triggering event. Earthquakes also release stored elastic potential energy in rocks, a store that has been produced ultimately from the same radioactive heat sources. Thus, according to present understanding, familiar events such as landslides and earthquakes release energy that has been stored as potential energy in the Earth's gravitational field or elastic strain (mechanical potential energy) in rocks. Prior to this, they represent release of energy that has been stored in heavy atoms since the collapse of long-destroyed supernova stars created these atoms.
Cosmology
In cosmology and astronomy the phenomena of stars, nova, supernova, quasars and gamma-ray bursts are the universe's highest-output energy transformations of matter. All stellar phenomena (including solar activity) are driven by various kinds of energy transformations. Energy in such transformations is either from gravitational collapse of matter (usually molecular hydrogen) into various classes of astronomical objects (stars, black holes, etc.), or from nuclear fusion (of lighter elements, primarily hydrogen). The nuclear fusion of hydrogen in the Sun also releases another store of potential energy which was created at the time of the Big Bang. At that time, according to theory, space expanded and the universe cooled too rapidly for hydrogen to completely fuse into heavier elements. This meant that hydrogen represents a store of potential energy that can be released by fusion. Such a fusion process is triggered by heat and pressure generated from gravitational collapse of hydrogen clouds when they produce stars, and some of the fusion energy is then transformed into sunlight.Quantum mechanics
In quantum mechanics, energy is defined in terms of the energy operator as a time derivative of the wave function. The Schrödinger equation equates the energy operator to the full energy of a particle or a system. Its results can be considered as a definition of measurement of energy in quantum mechanics. The Schrödinger equation describes the space- and time-dependence of a slowly changing (non-relativistic) wave function of quantum systems. The solution of this equation for a bound system is discrete (a set of permitted states, each characterized by an energy level) which results in the concept of quanta. In the solution of the Schrödinger equation for any oscillator (vibrator) and for electromagnetic waves in a vacuum, the resulting energy states are related to the frequency by Planck's relation: (where is Planck's constant and the frequency). In the case of an electromagnetic wave these energy states are called quanta of light or photons.
Relativity
When calculating kinetic energy (work to accelerate a massive body from zero speed to some finite speed) relativistically – using Lorentz transformations instead of Newtonian mechanics – Einstein discovered an unexpected by-product of these calculations to be an energy term which does not vanish at zero speed. He called it rest energy: energy which every massive body must possess even when being at rest. The amount of energy is directly proportional to the mass of the body:- ,
- m is the mass of the body,
- c is the speed of light in vacuum,
- is the rest energy.
In general relativity, the stress–energy tensor serves as the source term for the gravitational field, in rough analogy to the way mass serves as the source term in the non-relativistic Newtonian approximation.
Energy and mass are manifestations of one and the same underlying physical property of a system. This property is responsible for the inertia and strength of gravitational interaction of the system ("mass manifestations"), and is also responsible for the potential ability of the system to perform work or heating ("energy manifestations"), subject to the limitations of other physical laws.
In classical physics, energy is a scalar quantity, the canonical conjugate to time. In special relativity energy is also a scalar (although not a Lorentz scalar but a time component of the energy–momentum 4-vector). In other words, energy is invariant with respect to rotations of space, but not invariant with respect to rotations of space-time (= boosts).
Energy transformation
Type of transfer process | Description |
---|---|
Heat | that amount of thermal energy in transit spontaneously towards a lower-temperature object |
Work | that amount of energy in transit due to a displacement in the direction of an applied force |
Transfer of material | that amount of energy carried by matter that is moving from one system to another |

A turbo generator transforms the energy of pressurised steam into electrical energy
Examples of energy transformation include generating electric energy from heat energy via a steam turbine, or lifting an object against gravity using electrical energy driving a crane motor. Lifting against gravity performs mechanical work on the object and stores gravitational potential energy in the object. If the object falls to the ground, gravity does mechanical work on the object which transforms the potential energy in the gravitational field to the kinetic energy released as heat on impact with the ground. Our Sun transforms nuclear potential energy to other forms of energy; its total mass does not decrease due to that in itself (since it still contains the same total energy even if in different forms), but its mass does decrease when the energy escapes out to its surroundings, largely as radiant energy.
There are strict limits to how efficiently heat can be converted into work in a cyclic process, e.g. in a heat engine, as described by Carnot's theorem and the second law of thermodynamics. However, some energy transformations can be quite efficient. The direction of transformations in energy (what kind of energy is transformed to what other kind) is often determined by entropy (equal energy spread among all available degrees of freedom) considerations. In practice all energy transformations are permitted on a small scale, but certain larger transformations are not permitted because it is statistically unlikely that energy or matter will randomly move into more concentrated forms or smaller spaces.
Energy transformations in the universe over time are characterized by various kinds of potential energy that has been available since the Big Bang later being "released" (transformed to more active types of energy such as kinetic or radiant energy) when a triggering mechanism is available. Familiar examples of such processes include nuclear decay, in which energy is released that was originally "stored" in heavy isotopes (such as uranium and thorium), by nucleosynthesis, a process ultimately using the gravitational potential energy released from the gravitational collapse of supernovae, to store energy in the creation of these heavy elements before they were incorporated into the solar system and the Earth. This energy is triggered and released in nuclear fission bombs or in civil nuclear power generation. Similarly, in the case of a chemical explosion, chemical potential energy is transformed to kinetic energy and thermal energy in a very short time. Yet another example is that of a pendulum. At its highest points the kinetic energy is zero and the gravitational potential energy is at maximum. At its lowest point the kinetic energy is at maximum and is equal to the decrease of potential energy. If one (unrealistically) assumes that there is no friction or other losses, the conversion of energy between these processes would be perfect, and the pendulum would continue swinging forever.
Energy is also transferred from potential energy () to kinetic energy () and then back to potential energy constantly. This is referred to as conservation of energy. In this closed system, energy cannot be created or destroyed; therefore, the initial energy and the final energy will be equal to each other. This can be demonstrated by the following:
- (4)
Conservation of energy and mass in transformation
Energy gives rise to weight when it is trapped in a system with zero momentum, where it can be weighed. It is also equivalent to mass, and this mass is always associated with it. Mass is also equivalent to a certain amount of energy, and likewise always appears associated with it, as described in mass-energy equivalence. The formula E = mc², derived by Albert Einstein (1905) quantifies the relationship between rest-mass and rest-energy within the concept of special relativity. In different theoretical frameworks, similar formulas were derived by J. J. Thomson (1881), Henri Poincaré (1900), Friedrich Hasenöhrl (1904) and others (see Mass-energy equivalence#History for further information).Part of the rest energy (equivalent to rest mass) of matter may be converted to other forms of energy (still exhibiting mass), but neither energy nor mass can be destroyed; rather, both remain constant during any process. However, since is extremely large relative to ordinary human scales, the conversion of an everyday amount of rest mass (for example, 1 kg) from rest energy to other forms of energy (such as kinetic energy, thermal energy, or the radiant energy carried by light and other radiation) can liberate tremendous amounts of energy (~ joules = 21 megatons of TNT), as can be seen in nuclear reactors and nuclear weapons. Conversely, the mass equivalent of an everyday amount energy is minuscule, which is why a loss of energy (loss of mass) from most systems is difficult to measure on a weighing scale, unless the energy loss is very large. Examples of large transformations between rest energy (of matter) and other forms of energy (e.g., kinetic energy into particles with rest mass) are found in nuclear physics and particle physics.
Reversible and non-reversible transformations
Thermodynamics divides energy transformation into two kinds: reversible processes and irreversible processes. An irreversible process is one in which energy is dissipated (spread) into empty energy states available in a volume, from which it cannot be recovered into more concentrated forms (fewer quantum states), without degradation of even more energy. A reversible process is one in which this sort of dissipation does not happen. For example, conversion of energy from one type of potential field to another, is reversible, as in the pendulum system described above. In processes where heat is generated, quantum states of lower energy, present as possible excitations in fields between atoms, act as a reservoir for part of the energy, from which it cannot be recovered, in order to be converted with 100% efficiency into other forms of energy. In this case, the energy must partly stay as heat, and cannot be completely recovered as usable energy, except at the price of an increase in some other kind of heat-like increase in disorder in quantum states, in the universe (such as an expansion of matter, or a randomisation in a crystal).As the universe evolves in time, more and more of its energy becomes trapped in irreversible states (i.e., as heat or other kinds of increases in disorder). This has been referred to as the inevitable thermodynamic heat death of the universe. In this heat death the energy of the universe does not change, but the fraction of energy which is available to do work through a heat engine, or be transformed to other usable forms of energy (through the use of generators attached to heat engines), grows less and less.
Conservation of energy
The fact that energy can be neither created nor be destroyed is called the law of conservation of energy. In the form of the first law of thermodynamics, this states that a closed system's energy is constant unless energy is transferred in or out by work or heat, and that no energy is lost in transfer. The total inflow of energy into a system must equal the total outflow of energy from the system, plus the change in the energy contained within the system. Whenever one measures (or calculates) the total energy of a system of particles whose interactions do not depend explicitly on time, it is found that the total energy of the system always remains constant.While heat can always be fully converted into work in a reversible isothermal expansion of an ideal gas, for cyclic processes of practical interest in heat engines the second law of thermodynamics states that the system doing work always loses some energy as waste heat. This creates a limit to the amount of heat energy that can do work in a cyclic process, a limit called the available energy. Mechanical and other forms of energy can be transformed in the other direction into thermal energy without such limitations. The total energy of a system can be calculated by adding up all forms of energy in the system.
Richard Feynman said during a 1961 lecture:
There is a fact, or if you wish, a law, governing all natural phenomena that are known to date. There is no known exception to this law—it is exact so far as we know. The law is called the conservation of energy. It states that there is a certain quantity, which we call energy, that does not change in manifold changes which nature undergoes. That is a most abstract idea, because it is a mathematical principle; it says that there is a numerical quantity which does not change when something happens. It is not a description of a mechanism, or anything concrete; it is just a strange fact that we can calculate some number and when we finish watching nature go through her tricks and calculate the number again, it is the same.Most kinds of energy (with gravitational energy being a notable exception)[14] are subject to strict local conservation laws as well. In this case, energy can only be exchanged between adjacent regions of space, and all observers agree as to the volumetric density of energy in any given space. There is also a global law of conservation of energy, stating that the total energy of the universe cannot change; this is a corollary of the local law, but not vice versa.
This law is a fundamental principle of physics. As shown rigorously by Noether's theorem, the conservation of energy is a mathematical consequence of translational symmetry of time, a property of most phenomena below the cosmic scale that makes them independent of their locations on the time coordinate. Put differently, yesterday, today, and tomorrow are physically indistinguishable. This is because energy is the quantity which is canonical conjugate to time. This mathematical entanglement of energy and time also results in the uncertainty principle - it is impossible to define the exact amount of energy during any definite time interval. The uncertainty principle should not be confused with energy conservation - rather it provides mathematical limits to which energy can in principle be defined and measured.
Each of the basic forces of nature is associated with a different type of potential energy, and all types of potential energy (like all other types of energy) appears as system mass, whenever present. For example, a compressed spring will be slightly more massive than before it was compressed. Likewise, whenever energy is transferred between systems by any mechanism, an associated mass is transferred with it.
In quantum mechanics energy is expressed using the Hamiltonian operator. On any time scales, the uncertainty in the energy is by
In particle physics, this inequality permits a qualitative understanding of virtual particles which carry momentum, exchange by which and with real particles, is responsible for the creation of all known fundamental forces (more accurately known as fundamental interactions). Virtual photons (which are simply lowest quantum mechanical energy state of photons) are also responsible for electrostatic interaction between electric charges (which results in Coulomb law), for spontaneous radiative decay of exited atomic and nuclear states, for the Casimir force, for van der Waals bond forces and some other observable phenomena.
Energy transfer
Closed systems
Energy transfer can be considered for the special case of systems which are closed to transfers of matter. The portion of the energy which is transferred by conservative forces over a distance is measured as the work the source system does on the receiving system. The portion of the energy which does not do work during the transfer is called heat. Energy can be transferred between systems in a variety of ways. Examples include the transmission of electromagnetic energy via photons, physical collisions which transfer kinetic energy, and the conductive transfer of thermal energy.Energy is strictly conserved and is also locally conserved wherever it can be defined. In thermodynamics, for closed systems, the process of energy transfer is described by the first law:
- (1)
- (2)
Open systems
Beyond the constraints of closed systems, open systems can gain or lose energy in association with matter transfer (both of these process are illustrated by fueling an auto, a system which gains in energy thereby, without addition of either work or heat). Denoting this energy by , one may write- (3)
Thermodynamics
Internal energy
Internal energy is the sum of all microscopic forms of energy of a system. It is the energy needed to create the system. It is related to the potential energy, e.g., molecular structure, crystal structure, and other geometric aspects, as well as the motion of the particles, in form of kinetic energy. Thermodynamics is chiefly concerned with changes in internal energy and not its absolute value, which is impossible to determine with thermodynamics alone.First law of thermodynamics
The first law of thermodynamics asserts that energy (but not necessarily thermodynamic free energy) is always conserved[17] and that heat flow is a form of energy transfer. For homogeneous systems, with a well-defined temperature and pressure, a commonly used corollary of the first law is that, for a system subject only to pressure forces and heat transfer (e.g., a cylinder-full of gas) without chemical changes, the differential change in the internal energy of the system (with a gain in energy signified by a positive quantity) is given as- where the first term on the right is the heat transferred into the system, expressed in terms of temperature T and entropy S (in which entropy increases and the change dS is positive when the system is heated), and the last term on the right hand side is identified as work done on the system, where pressure is P and volume V (the negative sign results since compression of the system requires work to be done on it and so the volume change, dV, is negative when work is done on the system).
Equipartition of energy
The energy of a mechanical harmonic oscillator (a mass on a spring) is alternatively kinetic and potential. At two points in the oscillation cycle it is entirely kinetic, and at two points it is entirely potential. Over the whole cycle, or over many cycles, net energy is thus equally split between kinetic and potential. This is called equipartition principle; total energy of a system with many degrees of freedom is equally split among all available degrees of freedom.This principle is vitally important to understanding the behaviour of a quantity closely related to energy, called entropy. Entropy is a measure of evenness of a distribution of energy between parts of a system. When an isolated system is given more degrees of freedom (i.e., given new available energy states that are the same as existing states), then total energy spreads over all available degrees equally without distinction between "new" and "old" degrees. This mathematical result is called the second law of thermodynamics. The second law of thermodynamics is valid only for systems which are near or in equilibrium state. For non-equilibrium systems, the laws governing system’s behavior are still debatable. One of the guiding principles for these systems is the principle of maximum entropy production. It states that non equilibrium systems behave in such a way to maximize its entropy production
XXX . XXX 4%zero null 0 1 2 Energy transformation
Type of transfer process | Description |
---|---|
Heat | that amount of thermal energy in transit spontaneously towards a lower-temperature object |
Work | that amount of energy in transit due to a displacement in the direction of an applied force |
Transfer of material | that amount of energy carried by matter that is moving from one system to another |

A turbo generator transforms the energy of pressurised steam into electrical energy
Examples of energy transformation include generating electric energy from heat energy via a steam turbine, or lifting an object against gravity using electrical energy driving a crane motor. Lifting against gravity performs mechanical work on the object and stores gravitational potential energy in the object. If the object falls to the ground, gravity does mechanical work on the object which transforms the potential energy in the gravitational field to the kinetic energy released as heat on impact with the ground. Our Sun transforms nuclear potential energy to other forms of energy; its total mass does not decrease due to that in itself (since it still contains the same total energy even if in different forms), but its mass does decrease when the energy escapes out to its surroundings, largely as radiant energy.
There are strict limits to how efficiently heat can be converted into work in a cyclic process, e.g. in a heat engine, as described by Carnot's theorem and the second law of thermodynamics. However, some energy transformations can be quite efficient. The direction of transformations in energy (what kind of energy is transformed to what other kind) is often determined by entropy (equal energy spread among all available degrees of freedom) considerations. In practice all energy transformations are permitted on a small scale, but certain larger transformations are not permitted because it is statistically unlikely that energy or matter will randomly move into more concentrated forms or smaller spaces.
Energy transformations in the universe over time are characterized by various kinds of potential energy that has been available since the Big Bang later being "released" (transformed to more active types of energy such as kinetic or radiant energy) when a triggering mechanism is available. Familiar examples of such processes include nuclear decay, in which energy is released that was originally "stored" in heavy isotopes (such as uranium and thorium), by nucleosynthesis, a process ultimately using the gravitational potential energy released from the gravitational collapse of supernovae, to store energy in the creation of these heavy elements before they were incorporated into the solar system and the Earth. This energy is triggered and released in nuclear fission bombs or in civil nuclear power generation. Similarly, in the case of a chemical explosion, chemical potential energy is transformed to kinetic energy and thermal energy in a very short time. Yet another example is that of a pendulum. At its highest points the kinetic energy is zero and the gravitational potential energy is at maximum. At its lowest point the kinetic energy is at maximum and is equal to the decrease of potential energy. If one (unrealistically) assumes that there is no friction or other losses, the conversion of energy between these processes would be perfect, and the pendulum would continue swinging forever.
Energy is also transferred from potential energy () to kinetic energy () and then back to potential energy constantly. This is referred to as conservation of energy. In this closed system, energy cannot be created or destroyed; therefore, the initial energy and the final energy will be equal to each other. This can be demonstrated by the following:
- (4)
Conservation of energy and mass in transformation
Energy gives rise to weight when it is trapped in a system with zero momentum, where it can be weighed. It is also equivalent to mass, and this mass is always associated with it. Mass is also equivalent to a certain amount of energy, and likewise always appears associated with it, as described in mass-energy equivalence. The formula E = mc², derived by Albert Einstein (1905) quantifies the relationship between rest-mass and rest-energy within the concept of special relativity. In different theoretical frameworks, similar formulas were derived by J. J. Thomson (1881), Henri Poincaré (1900), Friedrich Hasenöhrl (1904) and others (see Mass-energy equivalence#History for further information).Part of the rest energy (equivalent to rest mass) of matter may be converted to other forms of energy (still exhibiting mass), but neither energy nor mass can be destroyed; rather, both remain constant during any process. However, since is extremely large relative to ordinary human scales, the conversion of an everyday amount of rest mass (for example, 1 kg) from rest energy to other forms of energy (such as kinetic energy, thermal energy, or the radiant energy carried by light and other radiation) can liberate tremendous amounts of energy (~ joules = 21 megatons of TNT), as can be seen in nuclear reactors and nuclear weapons. Conversely, the mass equivalent of an everyday amount energy is minuscule, which is why a loss of energy (loss of mass) from most systems is difficult to measure on a weighing scale, unless the energy loss is very large. Examples of large transformations between rest energy (of matter) and other forms of energy (e.g., kinetic energy into particles with rest mass) are found in nuclear physics and particle physics.
Reversible and non-reversible transformations
Thermodynamics divides energy transformation into two kinds: reversible processes and irreversible processes. An irreversible process is one in which energy is dissipated (spread) into empty energy states available in a volume, from which it cannot be recovered into more concentrated forms (fewer quantum states), without degradation of even more energy. A reversible process is one in which this sort of dissipation does not happen. For example, conversion of energy from one type of potential field to another, is reversible, as in the pendulum system described above. In processes where heat is generated, quantum states of lower energy, present as possible excitations in fields between atoms, act as a reservoir for part of the energy, from which it cannot be recovered, in order to be converted with 100% efficiency into other forms of energy. In this case, the energy must partly stay as heat, and cannot be completely recovered as usable energy, except at the price of an increase in some other kind of heat-like increase in disorder in quantum states, in the universe (such as an expansion of matter, or a randomisation in a crystal).As the universe evolves in time, more and more of its energy becomes trapped in irreversible states (i.e., as heat or other kinds of increases in disorder). This has been referred to as the inevitable thermodynamic heat death of the universe. In this heat death the energy of the universe does not change, but the fraction of energy which is available to do work through a heat engine, or be transformed to other usable forms of energy (through the use of generators attached to heat engines), grows less and less.
Conservation of energy
The fact that energy can be neither created nor be destroyed is called the law of conservation of energy. In the form of the first law of thermodynamics, this states that a closed system's energy is constant unless energy is transferred in or out by work or heat, and that no energy is lost in transfer. The total inflow of energy into a system must equal the total outflow of energy from the system, plus the change in the energy contained within the system. Whenever one measures (or calculates) the total energy of a system of particles whose interactions do not depend explicitly on time, it is found that the total energy of the system always remains constant.[11]While heat can always be fully converted into work in a reversible isothermal expansion of an ideal gas, for cyclic processes of practical interest in heat engines the second law of thermodynamics states that the system doing work always loses some energy as waste heat. This creates a limit to the amount of heat energy that can do work in a cyclic process, a limit called the available energy. Mechanical and other forms of energy can be transformed in the other direction into thermal energy without such limitations.[12] The total energy of a system can be calculated by adding up all forms of energy in the system.
Richard Feynman said during a 1961 lecture:[13]
There is a fact, or if you wish, a law, governing all natural phenomena that are known to date. There is no known exception to this law—it is exact so far as we know. The law is called the conservation of energy. It states that there is a certain quantity, which we call energy, that does not change in manifold changes which nature undergoes. That is a most abstract idea, because it is a mathematical principle; it says that there is a numerical quantity which does not change when something happens. It is not a description of a mechanism, or anything concrete; it is just a strange fact that we can calculate some number and when we finish watching nature go through her tricks and calculate the number again, it is the same.Most kinds of energy (with gravitational energy being a notable exception)[14] are subject to strict local conservation laws as well. In this case, energy can only be exchanged between adjacent regions of space, and all observers agree as to the volumetric density of energy in any given space. There is also a global law of conservation of energy, stating that the total energy of the universe cannot change; this is a corollary of the local law, but not vice versa.[12][13]
This law is a fundamental principle of physics. As shown rigorously by Noether's theorem, the conservation of energy is a mathematical consequence of translational symmetry of time,[15] a property of most phenomena below the cosmic scale that makes them independent of their locations on the time coordinate. Put differently, yesterday, today, and tomorrow are physically indistinguishable. This is because energy is the quantity which is canonical conjugate to time. This mathematical entanglement of energy and time also results in the uncertainty principle - it is impossible to define the exact amount of energy during any definite time interval. The uncertainty principle should not be confused with energy conservation - rather it provides mathematical limits to which energy can in principle be defined and measured.
Each of the basic forces of nature is associated with a different type of potential energy, and all types of potential energy (like all other types of energy) appears as system mass, whenever present. For example, a compressed spring will be slightly more massive than before it was compressed. Likewise, whenever energy is transferred between systems by any mechanism, an associated mass is transferred with it.
In quantum mechanics energy is expressed using the Hamiltonian operator. On any time scales, the uncertainty in the energy is by
In particle physics, this inequality permits a qualitative understanding of virtual particles which carry momentum, exchange by which and with real particles, is responsible for the creation of all known fundamental forces (more accurately known as fundamental interactions). Virtual photons (which are simply lowest quantum mechanical energy state of photons) are also responsible for electrostatic interaction between electric charges (which results in Coulomb law), for spontaneous radiative decay of exited atomic and nuclear states, for the Casimir force, for van der Waals bond forces and some other observable phenomena.
Energy transfer
Closed systems
Energy transfer can be considered for the special case of systems which are closed to transfers of matter. The portion of the energy which is transferred by conservative forces over a distance is measured as the work the source system does on the receiving system. The portion of the energy which does not do work during the transfer is called heat.[note 4] Energy can be transferred between systems in a variety of ways. Examples include the transmission of electromagnetic energy via photons, physical collisions which transfer kinetic energy,[note 5] and the conductive transfer of thermal energy.Energy is strictly conserved and is also locally conserved wherever it can be defined. In thermodynamics, for closed systems, the process of energy transfer is described by the first law:[note 6]
- (1)
- (2)
Open systems
Beyond the constraints of closed systems, open systems can gain or lose energy in association with matter transfer (both of these process are illustrated by fueling an auto, a system which gains in energy thereby, without addition of either work or heat). Denoting this energy by , one may write- (3)
Thermodynamics
Internal energy
Internal energy is the sum of all microscopic forms of energy of a system. It is the energy needed to create the system. It is related to the potential energy, e.g., molecular structure, crystal structure, and other geometric aspects, as well as the motion of the particles, in form of kinetic energy. Thermodynamics is chiefly concerned with changes in internal energy and not its absolute value, which is impossible to determine with thermodynamics alone.First law of thermodynamics
The first law of thermodynamics asserts that energy (but not necessarily thermodynamic free energy) is always conserved and that heat flow is a form of energy transfer. For homogeneous systems, with a well-defined temperature and pressure, a commonly used corollary of the first law is that, for a system subject only to pressure forces and heat transfer (e.g., a cylinder-full of gas) without chemical changes, the differential change in the internal energy of the system (with a gain in energy signified by a positive quantity) is given as- ,
This equation is highly specific, ignoring all chemical, electrical, nuclear, and gravitational forces, effects such as advection of any form of energy other than heat and pV-work. The general formulation of the first law (i.e., conservation of energy) is valid even in situations in which the system is not homogeneous. For these cases the change in internal energy of a closed system is expressed in a general form by
Equipartition of energy
The energy of a mechanical harmonic oscillator (a mass on a spring) is alternatively kinetic and potential. At two points in the oscillation cycle it is entirely kinetic, and at two points it is entirely potential. Over the whole cycle, or over many cycles, net energy is thus equally split between kinetic and potential. This is called equipartition principle; total energy of a system with many degrees of freedom is equally split among all available degrees of freedom.This principle is vitally important to understanding the behaviour of a quantity closely related to energy, called entropy. Entropy is a measure of evenness of a distribution of energy between parts of a system. When an isolated system is given more degrees of freedom (i.e., given new available energy states that are the same as existing states), then total energy spreads over all available degrees equally without distinction between "new" and "old" degrees. This mathematical result is called the second law of thermodynamics. The second law of thermodynamics is valid only for systems which are near or in equilibrium state. For non-equilibrium systems, the laws governing system’s behavior are still debatable. One of the guiding principles for these systems is the principle of maximum entropy production. It states that non equilibrium systems behave in such a way to maximize its entropy production
XXX . XXX 4% zero null 0 1 2 3 4 Transfer Energy
Spaceflight
Spaceflight (also written space flight) is ballistic flight into or through outer space. Spaceflight can occur with spacecraft with or without humans on board. Examples of human spaceflight include the U.S. Apollo Moon landing and Space Shuttle programs and the Russian Soyuz program, as well as the ongoing International Space Station. Examples of unmanned spaceflight include space probes that leave Earth orbit, as well as satellites in orbit around Earth, such as communications satellites. These operate either by telerobotic control or are fully autonomous.
Spaceflight is used in space exploration, and also in commercial activities like space tourism and satellite telecommunications. Additional non-commercial uses of spaceflight include space observatories, reconnaissance satellites and other Earth observation satellites.
A spaceflight typically begins with a rocket launch, which provides the initial thrust to overcome the force of gravity and propels the spacecraft from the surface of the Earth. Once in space, the motion of a spacecraft—both when unpropelled and when under propulsion—is covered by the area of study called astrodynamics. Some spacecraft remain in space indefinitely, some disintegrate during atmospheric reentry, and others reach a planetary or lunar surface for landing or impact.

Phase
Launch

Saturn V on the launch pad before the launch of Apollo 4
A launch is often restricted to certain launch windows. These windows depend upon the position of celestial bodies and orbits relative to the launch site. The biggest influence is often the rotation of the Earth itself. Once launched, orbits are normally located within relatively constant flat planes at a fixed angle to the axis of the Earth, and the Earth rotates within this orbit.
A launch pad is a fixed structure designed to dispatch airborne vehicles. It generally consists of a launch tower and flame trench. It is surrounded by equipment used to erect, fuel, and maintain launch vehicles.
Reaching space
The most commonly used definition of outer space is everything beyond the Kármán line, which is 100 kilometers (62 mi) above the Earth's surface. The United States sometimes defines outer space as everything beyond 50 miles (80 km) in altitude.Rockets are the only currently practical means of reaching space. Conventional airplane engines cannot reach space due to the lack of oxygen. Rocket engines expel propellant to provide forward thrust that generates enough delta-v (change in velocity) to reach orbit.
For manned launch systems launch escape systems are frequently fitted to allow astronauts to escape in the case of emergency.
Alternatives
Many ways to reach space other than rockets have been proposed. Ideas such as the space elevator, and momentum exchange tethers like rotovators or skyhooks require new materials much stronger than any currently known. Electromagnetic launchers such as launch loops might be feasible with current technology. Other ideas include rocket assisted aircraft/spaceplanes such as Reaction Engines Skylon (currently in early stage development), scramjet powered spaceplanes, and RBCC powered spaceplanes. Gun launch has been proposed for cargo.Leaving orbit
However, the parking orbit approach greatly simplified Apollo mission planning in several important ways. It substantially widened the allowable launch windows, increasing the chance of a successful launch despite minor technical problems during the countdown. The parking orbit was a stable "mission plateau" that gave the crew and controllers several hours to thoroughly check out the spacecraft after the stresses of launch before committing it to a long lunar flight; the crew could quickly return to Earth, if necessary, or an alternate Earth-orbital mission could be conducted. The parking orbit also enabled translunar trajectories that avoided the densest parts of the Van Allen radiation belts.
Apollo missions minimized the performance penalty of the parking orbit by keeping its altitude as low as possible. For example, Apollo 15 used an unusually low parking orbit (even for Apollo) of 92.5 nmi by 91.5 nmi (171 km by 169 km) where there was significant atmospheric drag. But it was partially overcome by continuous venting of hydrogen from the third stage of the Saturn V, and was in any event tolerable for the short stay.
Robotic missions do not require an abort capability or radiation minimization, and because modern launchers routinely meet "instantaneous" launch windows, space probes to the Moon and other planets generally use direct injection to maximize performance. Although some might coast briefly during the launch sequence, they do not complete one or more full parking orbits before the burn that injects them onto an Earth escape trajectory.
Note that the escape velocity from a celestial body decreases with altitude above that body. However, it is more fuel-efficient for a craft to burn its fuel as close to the ground as possible; see Oberth effect and reference. This is another way to explain the performance penalty associated with establishing the safe perigee of a parking orbit.
Plans for future crewed interplanetary spaceflight missions often include final vehicle assembly in Earth orbit, such as NASA's Project Orion and Russia's Kliper/Parom tandem.
Astrodynamics
Astrodynamics is the study of spacecraft trajectories, particularly as they relate to gravitational and propulsion effects. Astrodynamics allows for a spacecraft to arrive at its destination at the correct time without excessive propellant use. An orbital maneuvering system may be needed to maintain or change orbits.Non-rocket orbital propulsion methods include solar sails, magnetic sails, plasma-bubble magnetic systems, and using gravitational slingshot effects.
Ionized gas trail from Shuttle reentry
Recovery of Discoverer 14 return capsule by a C-119 airplane
Transfer energy
The term "transfer energy" means the total amount of energy imparted by a rocket stage to its payload. This can be the energy imparted by a first stage of a launch vehicle to an upper stage plus payload, or by an upper stage or spacecraft kick motor to a spacecraft.Reentry
Vehicles in orbit have large amounts of kinetic energy. This energy must be discarded if the vehicle is to land safely without vaporizing in the atmosphere. Typically this process requires special methods to protect against aerodynamic heating. The theory behind reentry was developed by Harry Julian Allen. Based on this theory, reentry vehicles present blunt shapes to the atmosphere for reentry. Blunt shapes mean that less than 1% of the kinetic energy ends up as heat that reaches the vehicle and the heat energy instead ends up in the atmosphere.Landing
The Mercury, Gemini, and Apollo capsules all splashed down in the sea. These capsules were designed to land at relatively low speeds with the help of a parachute. Russian capsules for Soyuz make use of a big parachute and braking rockets to touch down on land. The Space Shuttle glided to a touchdown like a plane.Recovery
After a successful landing the spacecraft, its occupants and cargo can be recovered. In some cases, recovery has occurred before landing: while a spacecraft is still descending on its parachute, it can be snagged by a specially designed aircraft. This mid-air retrieval technique was used to recover the film canisters from the Corona spy satellites.Types
Unmanned

The MESSENGER spacecraft at Mercury (artist's interpretation)
Unmanned space missions use remote-controlled spacecraft. The first unmanned space mission was Sputnik I, launched October 4, 1957 to orbit the Earth. Space missions where animals but no humans are on-board are considered unmanned missions.
Benefits
Many space missions are more suited to tele robotic rather than crewed operation, due to lower cost and lower risk factors. In addition, some planetary destinations such as Venus or the vicinity of Jupiter are too hostile for human survival, given current technology. Outer planets such as Saturn, Uranus, and Neptune are too distant to reach with current crewed spaceflight technology, so telerobotic probes are the only way to explore them. Telerobotics also allows exploration of regions that are vulnerable to contamination by Earth micro-organisms since spacecraft can be sterilized. Humans can not be sterilized in the same way as a spaceship, as they coexist with numerous micro-organisms, and these micro-organisms are also hard to contain within a spaceship or spacesuit.Telepresence
Telerobotics becomes telepresence when the time delay is short enough to permit control of the spacecraft in close to real time by humans. Even the two seconds light speed delay for the Moon is too far away for tele presence exploration from Earth. The L1 and L2 positions permit 400-millisecond round trip delays, which is just close enough for tele presence operation. Tele presence has also been suggested as a way to repair satellites in Earth orbit from Earth. The Exploration Tele robotics Symposium in 2012 explored this and other topics.Human

ISS crew member stores samples
Sub-orbital
On a sub-orbital spaceflight the spacecraft reaches space and then returns to the atmosphere after following a (primarily) ballistic trajectory. This is usually because of insufficient specific orbital energy, in which case a suborbital flight will last only a few minutes, but it is also possible for an object with enough energy for an orbit to have a trajectory that intersects the Earth's atmosphere, sometimes after many hours. Pioneer 1 was NASA's first space probe intended to reach the Moon. A partial failure caused it to instead follow a suborbital trajectory to an altitude of 113,854 kilometers (70,746 mi) before reentering the Earth's atmosphere 43 hours after launch.The most generally recognized boundary of space is the Kármán line 100 km above sea level. (NASA alternatively defines an astronaut as someone who has flown more than 50 miles (80 km) above sea level.) It is not generally recognized by the public that the increase in potential energy required to pass the Kármán line is only about 3% of the orbital energy (potential plus kinetic energy) required by the lowest possible Earth orbit (a circular orbit just above the Kármán line.) In other words, it is far easier to reach space than to stay there. On May 17, 2004, Civilian Space exploration Team launched the Go Fast Rocket on a suborbital flight, the first amateur spaceflight. On June 21, 2004, Space Ship One was used for the first privately funded human spaceflight.
Point-to-point
Point-to-point sub-orbital spaceflight is a category of spaceflight in which a spacecraft uses a sub-orbital flight for transportation. This can provide a two-hour trip from London to Sydney, which would be much faster than what is currently over a twenty-hour flight. Today, no company offers this type of spaceflight for transportation. However, Virgin Galactic has plans for a space plane called Space Ship Three, which could offer this service in the future. Suborbital spaceflight over an intercontinental distance requires a vehicle velocity that is only a little lower than the velocity required to reach low Earth orbit. If rockets are used, the size of the rocket relative to the payload is similar to an Intercontinental Ballistic Missile (ICBM). Any intercontinental spaceflight has to surmount problems of heating during atmosphere re-entry that are nearly as large as those faced by orbital spaceflight.Orbital
A minimal orbital spaceflight requires much higher velocities than a minimal sub-orbital flight, and so it is technologically much more challenging to achieve. To achieve orbital spaceflight, the tangential velocity around the Earth is as important as altitude. In order to perform a stable and lasting flight in space, the spacecraft must reach the minimal orbital speed required for a closed orbit.Interplanetary
Interplanetary travel is travel between planets within a single planetary system. In practice, the use of the term is confined to travel between the planets of our Solar System.Interstellar
Five spacecraft are currently leaving the Solar System on escape trajectories, Voyager 1, Voyager 2, Pioneer 10, Pioneer 11, and New Horizons. The one farthest from the Sun is Voyager 1, which is more than 100 AU distant and is moving at 3.6 AU per year.[12] In comparison, Proxima Centauri, the closest star other than the Sun, is 267,000 AU distant. It will take Voyager 1 over 74,000 years to reach this distance. Vehicle designs using other techniques, such as nuclear pulse propulsion are likely to be able to reach the nearest star significantly faster. Another possibility that could allow for human interstellar spaceflight is to make use of time dilation, as this would make it possible for passengers in a fast-moving vehicle to travel further into the future while aging very little, in that their great speed slows down the rate of passage of on-board time. However, attaining such high speeds would still require the use of some new, advanced method of propulsion.Intergalactic
Intergalactic travel involves spaceflight between galaxies, and is considered much more technologically demanding than even interstellar travel and, by current engineering terms, is considered science fiction.Spacecraft
Spacecraft are vehicles capable of controlling their trajectory through space.The first 'true spacecraft' is sometimes said to be Apollo Lunar Module, since this was the only manned vehicle to have been designed for, and operated only in space; and is notable for its non aerodynamic shape.
Propulsion
Spacecraft today predominantly use rockets for propulsion, but other propulsion techniques such as ion drives are becoming more common, particularly for unmanned vehicles, and this can significantly reduce the vehicle's mass and increase its delta-v.Launch systems
Launch systems are used to carry a payload from Earth's surface into outer space.Expendable
- All current spaceflight uses multi-stage expendable launch systems to reach space.
Reusable
- The first reusable spacecraft, the X-15, was air-launched on a suborbital trajectory on July 19, 1963. The first partially reusable orbital spacecraft, the Space Shuttle, was launched by the USA on the 20th anniversary of Yuri Gagarin's flight, on April 12, 1981. During the Shuttle era, six orbiters were built, all of which have flown in the atmosphere and five of which have flown in space. The Enterprise was used only for approach and landing tests, launching from the back of a Boeing 747 and gliding to deadstick landings at Edwards AFB, California. The first Space Shuttle to fly into space was the Columbia, followed by the Challenger, Discovery, Atlantis, and Endeavour. The Endeavour was built to replace the Challenger, which was lost in January 1986. The Columbia broke up during reentry in February 2003.
- The first automatic partially reusable spacecraft was the Buran (Snowstorm), launched by the USSR on November 15, 1988, although it made only one flight. This spaceplane was designed for a crew and strongly resembled the US Space Shuttle, although its drop-off boosters used liquid propellants and its main engines were located at the base of what would be the external tank in the American Shuttle. Lack of funding, complicated by the dissolution of the USSR, prevented any further flights of Buran.
- Per the Vision for Space Exploration, the Space Shuttle was retired in 2011 due mainly to its old age and high cost of the program reaching over a billion dollars per flight. The Shuttle's human transport role is to be replaced by the partially reusable Crew Exploration Vehicle (CEV) no later than 2021. The Shuttle's heavy cargo transport role is to be replaced by expendable rockets such as the Evolved Expendable Launch Vehicle (EELV) or a Shuttle Derived Launch Vehicle.
- Scaled Composites SpaceShipOne was a reusable suborbital spaceplane that carried pilots Mike Melvill and Brian Binnie on consecutive flights in 2004 to win the Ansari X Prize. The Spaceship Company will build its successor SpaceShipTwo. A fleet of Space Ship Twos operated by Virgin Galactic planned to begin reusable private spaceflight carrying paying passengers (space tourists) in 2008, but this was delayed due to an accident in the propulsion development.
Challenges
Space disasters
All launch vehicles contain a huge amount of energy that is needed for some part of it to reach orbit. There is therefore some risk that this energy can be released prematurely and suddenly, with significant effects. When a Delta II rocket exploded 13 seconds after launch on January 17, 1997, there were reports of store windows 10 miles (16 km) away being broken by the blast.Space is a fairly predictable environment, but there are still risks of accidental depressurization and the potential failure of equipment, some of which may be very newly developed.
In 2004 the International Association for the Advancement of Space Safety was established in the Netherlands to further international cooperation and scientific advancement in space systems safety.
Weightlessness

Astronauts on the ISS in weightless conditions. Michael Foale can be seen exercising in the foreground.
Radiation
Once above the atmosphere, radiation due to the Van Allen belts, solar radiation and cosmic radiation issues occur and increase. Further away from the Earth, solar flares can give a fatal radiation dose in minutes, and the health threat from cosmic radiation significantly increases the chances of cancer over a decade exposure or more.Life support
In human spaceflight, the life support system is a group of devices that allow a human being to survive in outer space. NASA often uses the phrase Environmental Control and Life Support System or the acronym ECLSS when describing these systems for its human spaceflight missions. The life support system may supply: air, water and food. It must also maintain the correct body temperature, an acceptable pressure on the body and deal with the body's waste products. Shielding against harmful external influences such as radiation and micro-meteorites may also be necessary. Components of the life support system are life-critical, and are designed and constructed using safety engineering techniques.Space weather
Space weather is the concept of changing environmental conditions in outer space. It is distinct from the concept of weather within a planetary atmosphere, and deals with phenomena involving ambient plasma, magnetic fields, radiation and other matter in space (generally close to Earth but also in interplanetary, and occasionally interstellar medium). "Space weather describes the conditions in space that affect Earth and its technological systems. Our space weather is a consequence of the behavior of the Sun, the nature of Earth's magnetic field, and our location in the Solar System."[19]Space weather exerts a profound influence in several areas related to space exploration and development. Changing geomagnetic conditions can induce changes in atmospheric density causing the rapid degradation of spacecraft altitude in Low Earth orbit. Geomagnetic storms due to increased solar activity can potentially blind sensors aboard spacecraft, or interfere with on-board electronics. An understanding of space environmental conditions is also important in designing shielding and life support systems for manned spacecraft.
Environmental considerations
Rockets as a class are not inherently grossly polluting. However, some rockets use toxic propellants, and most vehicles use propellants that are not carbon neutral. Many solid rockets have chlorine in the form of perchlorate or other chemicals, and this can cause temporary local holes in the ozone layer. Re-entering spacecraft generate nitrates which also can temporarily impact the ozone layer. Most rockets are made of metals that can have an environmental impact during their construction.In addition to the atmospheric effects there are effects on the near-Earth space environment. There is the possibility that orbit could become inaccessible for generations due to exponentially increasing space debris caused by spalling of satellites and vehicles (Kessler syndrome). Many launched vehicles today are therefore designed to be re-entered after use.
Applications

This shows an extreme ultraviolet view of the sun (the Apollo Telescope Mount SO82A Experiment) taken during Skylab 3, with the Earth added for scale. On the right an image of the Sun shows a helium emissions, and there is an image on the left showing emissions from iron. One application for spaceflight is to take observation hindered or made more difficult by being on Earth's surface. Skylab included a massive manned solar observatory that revolutionized solar science in the early 1970s using the Apollo-based space station in conjunction with manned spaceflights to it.
- Earth observation satellites such as Spy satellites, weather satellites
- Space exploration
- Communication satellites
- Satellite television
- Satellite navigation
- Space tourism
- Protecting Earth from Potentially hazardous objects
- Space colonization
Private spaceflight is a rapidly developing area: space flight that is not only paid for by corporations or even private individuals, but often provided by private spaceflight companies. These companies often assert that much of the previous high cost of access to space was caused by governmental inefficiencies they can avoid. This assertion can be supported by much lower published launch costs for private space launch vehicles such as Falcon 9 developed with private financing. Lower launch costs and excellent safety will be required for the applications such as Space tourism and especially Space colonization to become successful.
Tidak ada komentar:
Posting Komentar