Signal tracer To seek interference and damage to audio and video electronics circuits
Simple signal tracking circuit
PACO Z-80 signal tracer probe
The signal tracker consists of a detector (taking back the low frequency of the high frequency carrier) and an ear phone. This signal tracker is used to search for circuit breakage by using the ear - phone.
By
tracing the circuit from start-up to end-level by using a signal tracer
earphone, the principle works like a medical device that is a
stethoscope to examine the human body, with this signal tracker
detectable which circuit level is damaged, by drawing conclusions from
the inactivity of the earphone It, also this tracker can be used to look for damage to the sound circuit, where the test signal is disrupted.
When and how to use signal-tracing techniques ?
Xample signal tracing to simple amplifier :
By comparing the Vi and Vo readings, we can determine the strengthening. This method is also called Input-Output Method / Output-Input Method.
By
changing the output amplitudes of the signal generator, we can see
whether the amplifier is linear in the input signal region.
With the load impedance variation (RL), we can see whether the linear gain on the load changes.
By changing the frequency of the signal generator, we can determine the frequency response of the amplifier.
With
this simple arrangement, the important characteristics of the amplifier
can be measured by the signal-tracing system, at amplitude and
frequency, from input to amplifier output.
In
some electronic devices, external signaling is not always necessary,
especially when the signal that should exist on the equipment can be
easily known. This method is called passive signal-tracing method .
Xample Passive Tracing Signal Method A Power Supply :
The mesh voltage is measured with an AC voltmeter on the wall outlet, on the fuse, and on the switch. If there is a 220 V AC voltage at the primary end of the
transformer, then it is certain that the plug, cable, fuse and switch
are in good condition.
AC signal on the secondary of the transformer can be measured on each side (secondary of transformer no CT) to ground. If there is a voltage on the secondary transformer of appropriate
magnitude, then it can be ascertained that the transformer is in good
condition.
Next, use the meter switch on the DC scale. Measure the voltage on C1 and on C2. If there is no DC voltage on C1 or C2, it means that the capacitor is short-circuited. When the L winding is open, then there is only a DC voltage at C1, but not in C2. When
C1 and C2 are open (break), or when CR1 and CR2 directors are open, or
both are short-circuited, the measured DC voltage is incorrect. Under such conditions, it is necessary to measure resistance to ensure the damaged component.
The
second way is the opposite of the first way, ie starting from the
measurement of DC voltage on capacitor C2, followed by measurement of DC
voltage on capacitor C1 and so on. The result is the same because the measurement uses only voltmeter only.
The following example is an FM radio whose block diagram shown in figure does not work.
Examination of power supply and voltage at static state of the circuit has been done. The damage is in the area between the antenna and the audio amplifier. In the passive method, normal signals are considered to be present or known. However,
since the antenna and tuning (which are thought to give normal signals
to the system) are within the system itself, external signals must be
assigned as normal signals and use speakers as signal indicators. This method is called an active signal-tracing method or signal injection.
First step:
The signal generator is connected to the RF tuner, and the antenna is removed; The signal generator and tuner are set at the same frequency. If
you do not hear anything on the loudspeaker, move the signal generator
at point A. change the frequency of the generator signal at 10.7 MHz
(Standard for FM radio). When the sound is heard (tone of the generator signal), this means damage to the RF tuner.
If not heard something, move the generator signal to the amplifier
output, ie at point B. At this point, the amplitude of the generator
signal must be increased to compensate for the gain of the middle
amplifier.
At point C, the normal signal is an audio signal. Therefore, the signal generator entered through this point must be at the audio frequency.
At point D the generator signal should be strong enough to drive the loudspeaker. The
loudspeaker can be tested by checking the voltage on the amplifier
driver and minimizing it momentarily with the corresponding resistor
between voltage and ground. This should generate a click sound on the loudspeaker.
The second way:
The check is done from the speakers to the tuner. To
determine whether using the first or second way can be preliminary, for
example: by briefly amplifying the audio amplifier input with ground
using a screwdriver or the end of a clip, this should produce a
loudspeaker click (if the loudspeaker and audio amplifier are working
properly).
If you do not hear the sound, then the
second way is the best choice, because the damage must exist between the
loudspeaker and the audio amplifier.
When you hear
a click, you can still continue the examination in a second way from
point C, or in the first way, since both have the same chance of
checking speed.
Means:
The signal-tracing method requires input signals on the suspect area, and can be measured accurately. Signal-tracing always requires at least one test kit and generally two.
Methods of voltage and resistance
In general, voltage and resistance measurements are performed to check for suspected damaged tissue or components. Voltage measurements require equipment with ON conditions, whereas resistance measurements are made when the equipment is OFF.
Usually
circuit diagrams and datasheets indicate the voltage required for
normal operating conditions at a particular test point. By making such measurements, usually the location of damage to the network and components can be known.
Resistance measurement is a very useful method for checking electronics components. A simple resistance measurement can be used to ensure continuous
wiring, the correct value approach of the transformer, inductor, winding
as well as the value approach of the large capacitor.
The
majority of resistors used in electronic equipment are carbon
composition types and they tend to change in value due to age and heat. When
this happens often perhaps the measurement of resistor resistance or
other components in the circuit, should be assured by examination on the
circuit image. The parallel impedance does not give an incorrect measurement, when a
resistor increases in magnitude or open, it is relatively simple to
determine this.
Voltage and Barrier Techniques are often used anywhere after the
symptom-function technique refers to a particular circuit or component
as a source of damage, or when a signaltracing technique has localized a
defect in this way.
mean :Voltage
and Barrier methods are used to pinpoint a component or circuit
breakage and generally require company data for component and voltage
values.
The half-splitting method
This method is suitable for series with series blocks / open loop system (elongated) because it will be very fast when looking for damage. For example: function generator circuit, radio transmitter / receiver, etc.
The
step: starting from the center of the system, and successively on each
of the middle half of the part of the system that has been separated
until it is found to be damaged.
Block 1 ---> block 2 ---> block 3 ---->block 4 ----->block 5 ----> block 6 ----> block 7 ----> block8
As an insight
material that in the electronics block diagram shows the signal flow,
while the schematic circuit shows the flow of current and voltage, flow
chart is the flow of the program or function.
8 Block Sub Serial System Series
Check the output of block 4, if it works fine, means blocks 1 through 4 there is no problem.
If it does not work, then check block 2 output (middle of blocks
1-4), and if good means check out block 3 output, and if good means
block 4 is damaged.
Check Output of block 8, if it works well, means block 5 to block 8 is no problem.
If it does not work, then check the output of block 6 (middle of
blocks 1-4), and if good means check out the output of block 7, and if
good, then block 8 is damaged.
Loop breaking method
Electronic systems or sub-systems with feedback, very difficult to trace the damage without breaking the loop. Appropriate DC voltage or signal, shall be injected at the point where the loop is disconnected.
Voltages and signals through the circuit, should be used to monitor errors.
Inverted voltage or signal can be changed, to see the change of circuit response from normal state.
Typically, the loop is broken at the point where the signal is at small power, so it can be injected properly.
This technique can be used for example on a PLL (phase lock loop), such as :
The power supply and reference oscillator output should be checked first before the loop is disconnected. In this case the output should be abnormal or unstable or missing, so we can be sure that the VCO is not good. Next we can do the loop termination at the appropriate point. So
the loop termination here is not necessarily the feedback section, but
is sought in small signal areas that are easily injected with existing
equipment.
Substitution method
In this method it is usually necessary to sold or replace parts as the final stage of the damage tracking process.
There
are two main stages in the substitution method that must be done,
namely: use the correct replacement components, and connect correctly to
the circuit.
Prior to the replacement, it
is advisable to check with other methods as described previously, to be
sure which component is damaged.
Do a voltage measurement to make sure that the voltage that is supposed to exist really exists. The
voltage checks performed on the combined components of resistors and
capacitors, will be able to show whether they are damaged or just one of
them.
In practice, we are usually very difficult to
find a replacement component in the form of ICs, transistors, and diodes
that exactly match the replaced components. To solve this, you need to find equivalent data of IC type, transistor, or diode in semiconductor user manual.
If
the replaced component has a special type, for example: transformer,
coil-deflection yoke, and other special components, it is necessary to
look for a completely suitable replacement component (no equivalent).
PRINCIPLE OF DAMAGE TRACK / FAILURE
1. Maintenance Process in Industry
2. Specifications
3. Reliability and Failure
4. Method-Tracking Method of Damage
5. Problem-Solving Analysis
6. Active Component Testing
7. Circuit Checking and Testing
X . I
Detector (radio) / tracker

In
electronics, a
detector is an older term for an
electronic component in a
radio receiver that extracts
information contained in a
modulated radio wave, a
demodulator. The term dates from the first three decades of radio (1886-1916). Unlike modern radio stations which transmit sound (an
audio signal) on the radio
carrier wave, the first radio transmitters transmitted information by
wireless telegraphy, using different length pulses of radio waves to spell out text messages in
Morse code.
So early radio receivers did not have to extract an audio signal
(sound) from the incoming radio signal, but only detect the presence or
absence of the radio signal, to produce clicks in the receiver's
earphones representing the Morse code symbols. The device that did this
was called a
detector. A variety of different detector devices, such as the
coherer,
electrolytic detector, and
magnetic detector, were used during the wireless telegraphy era.
After sound (
amplitude modulation, AM) transmission began around 1920, the term evolved to mean a
demodulator, a nonlinear
rectifier (usually a
crystal diode or a
vacuum tube) which extracted the
audio signal from the radio frequency
carrier wave. This is its current meaning, although modern detectors usually consist of
semiconductor diodes,
transistors, or
integrated circuits.
In a
superheterodyne receiver the term is also sometimes used to refer to the
mixer, the tube or transistor which converts the incoming radio frequency signal to the
intermediate frequency. The mixer is called the
first detector, while the demodulator that extracts the audio signal from the intermediate frequency is called the
second detector.
A coherer detector, useful only for Morse code signals.
Amplitude modulation detectors
Envelope detector
A simple envelope detector
A simple crystal radio with no tuned circuit can be used to listen to strong AM broadcast signals
One major technique is known as envelope detection. The simplest form of
envelope detector is the diode detector that consists of a
diode
connected between the input and output of the circuit, with a resistor
and capacitor in parallel from the output of the circuit to the ground
to form a low pass filter. If the resistor and capacitor are correctly
chosen, the output of this circuit will be a nearly identical
voltage-shifted version of the original signal.
An early form of envelope detector was the
cat's whisker, which was used in the
crystal set
radio receiver. A later version using a crystal diode is still used in
crystal radio sets today. The limited frequency response of the headset
eliminates the RF component, making the low pass filter unnecessary.
More sophisticated envelope detectors include the
plate detector,
grid-leak detector and transistor equivalents of them,
infinite-impedance detectors (
peak detector circuits), and
precision rectifiers.
Product detector
A
product detector is a type of
demodulator used for
AM and
SSB signals, where the original carrier signal is removed by multiplying the received signal with a signal at the
carrier frequency
(or near to it). Rather than converting the envelope of the signal into
the decoded waveform by rectification as an envelope detector would,
the product detector takes the product of the modulated signal and a
local oscillator, hence the name. By
heterodyning,
the received signal is mixed (in some type of nonlinear device) with a
signal from the local oscillator, to give sum and difference frequencies
to the signals being mixed, just as a
first mixer stage in a
superhet would produce an
intermediate frequency; the
beat frequency in this case, the low frequency
modulating signal is recovered and the unwanted high frequencies
filtered out from the output of the product detector.
Product detector circuits are
analog multipliers and so essentially
ring modulators or
synchronous detectors and closely related to some
phase-sensitive detector circuits. They can be implemented using something as simple as
ring of diodes or a single dual-gate
Field Effect Transistor to anything as sophisticated as an
Integrated Circuit containing a
Gilbert cell.
Frequency and phase modulation detectors
AM detectors cannot demodulate
FM and
PM signals because both have a
constant amplitude. However an AM radio may detect the sound of an FM broadcast by the phenomenon of
slope detection
which occurs when the radio is tuned slightly above or below the
nominal broadcast frequency. Frequency variation on one sloping side of
the radio tuning curve gives the amplified signal a corresponding local
amplitude variation, to which the AM detector is sensitive. Slope
detection gives inferior distortion and noise rejection compared to the
following dedicated FM detectors that are normally used.
Phase detector
A
phase detector is a
nonlinear device whose output represents the
phase
difference between the two oscillating input signals. It has two inputs
and one output: a reference signal is applied to one input and the
phase or frequency modulated signal is applied to the other. The output
is a signal that is proportional to the phase difference between the two
inputs.
In phase demodulation the information is contained in the amount and rate of phase shift in the
carrier wave.
The Foster-Seeley discriminator
The
Foster-Seeley discriminator is a widely used FM detector. The detector consists of a special center-tapped
transformer feeding two diodes in a full wave DC
rectifier
circuit. When the input transformer is tuned to the signal frequency,
the output of the discriminator is zero. When there is no deviation of
the carrier, both halves of the center tapped transformer are balanced.
As the FM signal swings in frequency above and below the carrier
frequency, the balance between the two halves of the center-tapped
secondary is destroyed and there is an output voltage proportional to
the frequency deviation.
Ratio detector
A ratio detector using solid-state diodes
The ratio detector
is a variant of the Foster-Seeley discriminator, but one diode conducts
in an opposite direction, and using a tertiary winding in the preceding
transformer. The output in this case is taken between the sum of the
diode voltages and the center tap. The output across the diodes is
connected to a large value capacitor, which eliminates AM noise in the
ratio detector output. The ratio detector has the advantage over the
Foster-Seeley discriminator that it will not respond to
AM signals,
thus potentially saving a limiter stage; however the output is only 50%
of the output of a discriminator for the same input signal. The ratio
detector has wider bandwidth but more distortion than the Foster-Seeley
discriminator.
Quadrature detector
In quadrature detectors, the received FM signal is split into two signals. One of the two signals is then passed through a
high-reactance capacitor,
which shifts the phase of that signal by 90 degrees. This phase-shifted
signal is then applied to an LC circuit, which is resonant at the FM
signal's unmodulated, "center," or "carrier" frequency. If the received
FM signal's frequency equals the center frequency, then the two signals
will have a 90-degree
phase difference
and they are said to be in "phase quadrature" — hence the name of this
method. The two signals are then multiplied together in an analog or
digital device, which serves as a
phase detector;
that is, a device whose output is proportional to the phase difference
between two signals. In the case of an unmodulated FM signal, the phase
detector's output is — after the output has been
filtered;
that is, averaged over time — constant; namely, zero. However, if the
received FM signal has been modulated, then its frequency will vary from
the center frequency. In this case, the resonant LC circuit will
further shift the phase of the signal from the capacitor, so that the
signal's total phase shift will be the sum of the 90 degrees that's
imposed by the capacitor and the positive or negative phase change
that's imposed by the LC circuit. Now the output from the phase detector
will differ from zero, and in this way, one recovers the original
signal that was used to modulate the FM carrier.
This detection process can also be accomplished by combining, in an
exclusive-OR (XOR) logic gate, the original FM signal and a
square wave
whose frequency equals the FM signal's center frequency. The XOR gate
produces an output pulse whose duration equals the difference between
the times at which the square wave and the received FM signal pass
through zero volts. As the FM signal's frequency varies from its
unmodulated center frequency (which is also the frequency of the square
wave), the output pulses from the XOR gate become longer or shorter. (In
essence, this quadrature detector converts an FM signal into a
pulse-width modulated
(PWM) signal.) When these pulses are filtered, the filter's output
rises as the pulses grow longer and its output falls as the pulses grow
shorter. In this way, one recovers the original signal that was used to
modulate the FM carrier.
Other FM detectors
Less common, specialized, or obsolescent types of detectors include:
- Travis or double tuned circuit discriminator using two non-interacting tuned circuits above and below the nominal center frequency
- Weiss discriminator which uses a single LC tuned circuit or crystal
- Pulse count discriminator which converts the frequency to a train of
constant amplitude pulses, producing a voltage directly proportional to
the frequency.
Phase-locked loop detector
The
phase-locked loop detector requires no frequency-selective LC network to accomplish demodulation. In this system, a
voltage controlled oscillator (VCO) is
phase locked by a
feedback loop,
which forces the VCO to follow the frequency variations of the incoming
FM signal. The low-frequency error voltage that forces the VCO's
frequency to track the frequency of the modulated FM signal is the
demodulated audio output.
X . II
CRYSTAL RADIO AND DETEKTOR
A
crystal radio receiver, also called a
crystal set or
cat's whisker receiver, is a very simple
radio receiver, popular in the early days of radio. It needs no other power source but that received solely from the power of
radio waves received by a wire
antenna. It gets its name from its most important component, known as a
crystal detector, originally made from a piece of crystalline mineral such as
galena. This component is now called a
diode.
Crystal radios are the simplest type of radio receiver an
Tutup d can be made with a few inexpensive parts, such as a wire for an antenna, a
coil of copper wire for adjustment, a capacitor, a crystal detector, and
earphones. Crystal radios are distinct from ordinary radios as they are
passive receivers, while other radios use a separate source of
electric power such as a
battery or the
mains power to
amplify
the weak radio signal so as to make it louder. Thus, crystal sets
produce rather weak sound and must be listened to with sensitive
earphones, and can only receive stations within a limited range.
Today they
are still sold as educational devices, and there are groups of
enthusiasts devoted to their construction
.
Crystal radios receive
amplitude modulated (AM) signals, and can be designed to receive almost any
radio frequency band, but most receive the
AM broadcast band. A few receive
shortwave bands, but strong signals are required. The first crystal sets received
wireless telegraphy signals broadcast by
spark-gap transmitters at frequencies as low as 20 kHz.

1970s-era crystal radio marketed to children. The earphone is on left.
The antenna wire, right, has a clip to attach to metal objects such as a
bedspring, which serve as an additional antenna to improve reception.
Bright clouds
A family listening to a crystal radio in the 1920s
Greenleaf Whittier Pickard's US Patent 836,531 "Means for receiving intelligence communicated by electric waves" diagram
Radio receiver, Basel, Switzerland, 1914
NBS Circular 120 Home Crystal Radio Project
Crystal radio used as a backup receiver on a World War II
Liberty ship
Crystal radio was invented by a long, partly obscure chain of
discoveries
in the late 19th century that gradually evolved into more and more
practical radio receivers in the early 20th century. The earliest
practical use of crystal radio was to receive
Morse code radio signals transmitted, from
spark-gap transmitters, by early
amateur radio
experimenters. As electronics evolved, the ability to send voice
signals by radio caused a technological explosion in the years around
1920 that evolved into today's radio
broadcasting industry.
Early years
Early radio telegraphy used
spark gap and
arc transmitters as well as
high-frequency alternators running at
radio frequencies. The
coherer was the first means of detecting a radio signal. These, however, lacked the sensitivity to detect weak signals.
In the early 20th century, various researchers discovered that certain metallic
minerals, such as
galena, could be used to detect radio signals.
Pickard's detector was revolutionary in that he found that a fine pointed wire known as a "
cat's whisker", in delicate contact with a mineral, produced the best semiconductor effect (that of
rectification).
A crystal detector includes a crystal, a special thin wire that
contacts the crystal, and the stand that holds those components in
place. The most common crystal used is a small piece of
galena;
pyrite
was also often used, as it was a more easily adjusted and stable
mineral, and quite sufficient for urban signal strengths. Several other
minerals also performed well as detectors. Another benefit of crystals
was that they could
demodulate amplitude modulated signals.
This device brought
radiotelephones and
voice broadcast
to a public audience. Crystal sets represented an inexpensive and
technologically simple method of receiving these signals at a time when
the embryonic radio broadcasting industry was beginning to grow.
Crystodyne
crystodyne applying voltage
biases to various kinds of crystals for manufacture of radio detectors. The result was astonishing: with a
zincite (
zinc oxide) crystal he gained amplification. This was
negative resistance phenomenon, decades before the development of the
tunnel diode. After the first experiments, Losev built regenerative and
superheterodyne receivers, and even transmitters.
A crystodyne could be produced in primitive conditions; it can be made in a rural forge, unlike
vacuum tubes
and modern semiconductor devices. However, this discovery was not
supported by authorities and soon forgotten; no device was produced in
mass quantity beyond a few examples for research.
"Foxhole radios"
"Foxhole radio" used on the Italian Front in World War 2. It uses a
pencil lead attached to a safety pin pressing against a razor blade for a
detector.
In addition to mineral crystals, the oxide coatings of many metal surfaces act as
semiconductors
(detectors) capable of rectification. Crystal radios have been
improvised using detectors made from rusty nails, corroded pennies, and
many other common objects.
Design
Block diagram of a crystal radio receiver
A crystal radio can be thought of as a radio receiver reduced to its essentials. It consists of at least these components:
- An antenna in which electric currents are induced by the radio waves.
- A resonant circuit (tuned circuit) which serves to select the frequency of the desired radio station out of all the radio signals received by the antenna. The tuned circuit consists of a coil of wire (called an inductor) and a capacitor
connected together, so as to create a circuit that resonates at the
frequency of the desired station, and hence "tune" in that station. One
or both of the coil or capacitor is adjustable, allowing the circuit to
be tuned to different frequencies. In some circuits a capacitor is not
used, as the antenna also serves as the capacitor. The tuned circuit has
a resonant frequency
and allows radio waves at that frequency to pass to the detector, while
rejecting waves at all other frequencies. Such a circuit is also known
as a bandpass filter.
- A semiconductor crystal (detector) that demodulates the radio signal to get the audio signal (modulation). The crystal detector is a nonlinear impedance that functions as a square law detector[citation needed]. The detector's output is converted to sound by the earphone. Early sets used a cat's whisker detector, consisting of a fine wire touching the surface of a sample of crystalline mineral such as galena. It was this component that gave crystal sets their name.
- An earphone
to convert the audio signal to sound waves so they can be heard. The
low power produced by a crystal receiver is insufficient to power a loudspeaker, hence earphones are used.
Pictorial diagram from 1922 showing the circuit of a crystal radio. This common circuit did not use a tuning
capacitor, but used the capacitance of the antenna to form the
tuned circuit
with the coil. The detector might have been a piece of galena with a
whisker wire in contact with it on a part of the crystal, making a diode
contact
As a crystal radio has no power supply, the sound power produced by the earphone comes solely from the
transmitter of the radio station being received, via the radio waves captured by the antenna. The power available to a receiving antenna decreases with the square of its distance from the
radio transmitter. Even for a powerful commercial
broadcasting station, if it is more than a few miles from the receiver the power received by the antenna is very small, typically measured in
microwatts or
nanowatts. In modern crystal sets, signals as weak as 50
picowatts at the antenna can be heard. Crystal radios can receive such weak signals without using
amplification only due to the great sensitivity of human
hearing, which can detect sounds with an intensity of only 10
−16 W/cm
2.
Therefore, crystal receivers have to be designed to convert the energy
from the radio waves into sound waves as efficiently as possible. Even
so, they are usually only able to receive stations within distances of
about 25 miles for
AM broadcast stations, although the
radiotelegraphy signals used during the
wireless telegraphy era could be received at hundreds of miles, and crystal receivers were even used for transoceanic communication during that period.
Commercial passive receiver development was abandoned with the advent
of reliable vacuum tubes around 1920, and subsequent crystal radio
research was primarily done by
radio amateurs and hobbyists.
Many different circuits have been used. The following sections discuss the parts of a crystal radio in greater detail.
Antenna
The antenna converts the energy in the electromagnetic
radio waves to an
alternating electric current
in the antenna, which is connected to the tuning coil. Since in a
crystal radio all the power comes from the antenna, it is important that
the antenna collect as much power from the radio wave as possible. The
larger an antenna, the more power it can intercept. Antennas of the type
commonly used with crystal sets are most effective when their length is
close to a multiple of a quarter-
wavelength of the radio waves they are receiving. Since the length of the waves used with crystal radios is very long (
AM broadcast band waves are 182-566
m or 597–1857 ft. long) the antenna is made as long as possible, from a
long wire, in contrast to the
whip antennas or ferrite
loopstick antennas used in modern radios.
Serious crystal radio hobbyists use "inverted L" and
"T" type antennas,
consisting of hundreds of feet of wire suspended as high as possible
between buildings or trees, with a feed wire attached in the center or
at one end leading down to the receiver.
However more often random lengths of wire dangling out windows are
used. A popular practice in early days (particularly among apartment
dwellers) was to use existing large metal objects, such as
bedsprings,
fire escapes, and
barbed wire fences as antennas.
Ground
The wire antennas used with crystal receivers are
monopole antennas which develop their output voltage with respect to ground. The receiver thus requires a connection to
ground
(the earth) as a return circuit for the current. The ground wire was
attached to a radiator, water pipe, or a metal stake driven into the
ground. In early days if an adequate ground connection could not be made a
counterpoise was sometimes used. A good ground is more important for crystal sets than it is for powered receivers, as crystal sets are designed to have a low
input impedance
needed to transfer power efficiently from the antenna. A low resistance
ground connection (preferably below 25 Ω) is necessary because any
resistance in the ground reduces available power from the antenna.
In contrast, modern receivers are voltage-driven devices, with high
input impedance, hence little current flows in the antenna/ground
circuit. Also,
mains powered
receivers are grounded adequately through their power cords, which are
in turn attached to the earth by way of a well established ground.
Tuned circuit
The earliest crystal receiver circuit did not have a
tuned circuit
The
tuned circuit, consisting of a coil and a
capacitor connected together, acts as a
resonator, similar to a tuning fork.
Electric charge, induced in the antenna by the radio waves, flows
rapidly back and forth between the plates of the capacitor through the
coil. The circuit has a high
impedance at the desired radio signal's frequency, but a low impedance at all other frequencies.
Hence, signals at undesired frequencies pass through the tuned circuit
to ground, while the desired frequency is instead passed on to the
detector (diode) and stimulates the earpiece and is heard. The frequency
of the station received is the
resonant frequency f of the tuned circuit, determined by the
capacitance C of the capacitor and the
inductance L of the coil:

By varying either the inductor (L) or the capacitance (C), the
circuit can be adjusted to different frequencies. In inexpensive sets,
the inductor was made variable via a spring contact pressing against the
windings that could slide along the coil, thereby introducing a larger
or smaller number of turns of the coil into the circuit. Thus the
inductance could be varied, "tuning" the circuit to the frequencies of different radio stations.Alternatively, a
variable capacitor is used to tune the circuit. Some modern crystal sets use a
ferrite core tuning coil, in which a ferrite
magnetic core is moved into and out of the coil, thereby varying the inductance by changing the
magnetic permeability (this eliminated the less reliable mechanical contact).
The antenna is an integral part of the tuned circuit and its
reactance contributes to determining the circuit's resonant frequency. Antennas usually act as a
capacitance, as antennas shorter than a quarter-wavelength have
capacitive reactance. Many early crystal sets did not have a tuning capacitor, and relied instead on the capacitance inherent in the wire antenna (in addition to significant
parasitic capacitance in the coil) to form the tuned circuit with the coil.
The earliest crystal receivers did not have a tuned circuit at all,
and just consisted of a crystal detector connected between the antenna
and ground, with an earphone across it. Since this circuit lacked any frequency-selective elements besides the broad
resonance
of the antenna, it had little ability to reject unwanted stations, so
all stations within a wide band of frequencies were heard in the
earphone
(in practice the most powerful usually drowns out the others). It was
used in the earliest days of radio, when only one or two stations were
within a crystal set's limited range.
Impedance matching
"Two

slider" crystal radio circuit.
and example from 1920s. The two sliding contacts on the coil allowed
the impedance of the radio to be adjusted to match the antenna as theradio was tuned, resulting in stronger reception
An important principle used in crystal radio design to transfer maximum power to the earphone is
impedance matching. The maximum power is transferred from one part of a circuit to another when the
impedance of one circuit is the complex conjugate of that of the other; this implies that the two circuits should have equal resistance. However, in crystal sets, the impedance of the antenna-ground system (around 10-200
ohms ) is usually lower than the impedance of the receiver's tuned circuit (thousands of ohms at resonance),
and also varies depending on the quality of the ground attachment,
length of the antenna, and the frequency to which the receiver is tuned.
Therefore, in improved receiver circuits, in order to match the
antenna impedance to the receiver's impedance, the antenna was connected
across only a portion of the tuning coil's turns. This made the tuning coil act as an
impedance matching transformer (in an
autotransformer
connection) in addition to providing the tuning function. The antenna's
low resistance was increased (transformed) by a factor equal to the
square of the turns ratio (the ratio of the number of turns the antenna
was connected to, to the total number of turns of the coil), to match
the resistance across the tuned circuit.
In the "two-slider" circuit, popular during the wireless era, both the
antenna and the detector circuit were attached to the coil with sliding
contacts, allowing (interactive) adjustment of both the resonant frequency and the turns ratio.
Alternatively a multiposition switch was used to select taps on the
coil. These controls were adjusted until the station sounded loudest in
the earphone.
Problem of selectivity
Direct-coupled circuit with impedance matching
One of the drawbacks of crystal sets is that they are vulnerable to interference from stations near in
frequency to the desired station; that is to say, they have low
selectivity.
Often two or more stations are heard simultaneously. This is because
the simple tuned circuit does not reject nearby signals well; it allows a
wide band of frequencies to pass through, that is, it has a large
bandwidth (low
Q factor) compared to modern receivers.
The crystal detector worsened the problem, because it has relatively low
resistance, thus it "loaded" the tuned circuit
, damping the oscillations (lowering the response), and reducing its
Q.
In many circuits, the selectivity was improved by connecting the
detector and earphone circuit to a tap across only a fraction of the
coil's turns. This reduced the impedance loading of the tuned circuit, as well as improving the impedance match with the detector.
Inductive coupling
Inductively-coupled circuit with impedance matching. This type was used in most quality crystal receivers
Amateur-built crystal receiver with "loose coupler" antenna transformer, Belfast, around 1914
In more sophisticated crystal receivers, the tuning coil is replaced with an adjustable air core
antenna coupling transformer which improves the
selectivity by a technique called
loose coupling.
This consists of two
magnetically coupled coils of wire, one (the
primary) attached to the antenna and ground and the other (the
secondary)
attached to the rest of the circuit. The current from the antenna
creates an alternating magnetic field in the primary coil, which induced
a current in the secondary coil which was then rectified and powered
the earphone. Each of the coils functions as a
tuned circuit; the primary coil
resonated
with the capacitance of the antenna (or sometimes another capacitor),
and the secondary coil resonated with the tuning capacitor. Both the
primary and secondary were tuned to the frequency of the station. The
two circuits interacted to form a
resonant transformer.
Reducing the
coupling between the coils, by physically separating them so that less of the
magnetic field of one intersects the other, reduces the
mutual inductance, narrows the bandwidth, and results in much sharper, more selective tuning than that produced by a single tuned circuit.
However, the looser coupling also reduced the power of the signal
passed to the second circuit. The transformer was made with adjustable
coupling, to allow the listener to experiment with various settings to
gain the best reception.
One design common in early days, called a "loose coupler", consisted of a smaller secondary coil inside a larger primary coil
. The smaller coil was mounted on a
rack
so it could be slid linearly in or out of the larger coil. If radio
interference was encountered, the smaller coil would be slid further out
of the larger, loosening the coupling, narrowing the bandwidth, and
thereby rejecting the interfering signal.
The antenna coupling transformer also functioned as an
impedance matching transformer,
that allowed a better match of the antenna impedance to the rest of the
circuit. One or both of the coils usually had several taps which could
be selected with a switch, allowing adjustment of the number of turns of
that transformer and hence the "turns ratio".
Coupling transformers were difficult to adjust, because the three
adjustments, the tuning of the primary circuit, the tuning of the
secondary circuit, and the coupling of the coils, were all interactive,
and changing one affected the others.
Crystal detector
Galena cat's whisker detector
How the crystal detector works.
(A) The
amplitude modulated radio signal from the tuned circuit. The rapid oscillations are the
radio frequency carrier wave. The
audio signal (the sound) is contained in the slow variations (
modulation)
of the amplitude (hence the term amplitude modulation, AM) of the
waves. This signal cannot be converted to sound by the earphone, because
the audio excursions are the same on both sides of the axis, averaging
out to zero, which would result in no net motion of the earphone's
diaphragm.
(B) The crystal
conducts current better in one direction than the other, producing a
signal whose amplitude does not average to zero but varies with the
audio signal.
(C) A bypass capacitor is used to remove the radio frequency carrier pulses, leaving the audio signal
Circuit with detector bias battery to improve sensitivity and buzzer to adjust cat's whisker
The crystal
detector demodulates the radio frequency signal, extracting the
modulation (the
audio signal which represents the sound waves) from the radio frequency
carrier wave. In early receivers, the detector was a
cat's whisker detector consisting of a fine metal wire, the "cat's whisker", on an adjustable arm that touched a pea-sized lump of
semiconducting mineral. The point of contact between the wire and the crystal produced a diode effect. The cat's whisker detector was a crude
Schottky diode that allowed current to flow better in one direction than in the opposite direction. Modern crystal sets use modern
semiconductor diodes. The crystal functions as an
envelope detector,
rectifying the
alternating current radio signal to a pulsing
direct current,
the peaks of which trace out the audio signal, so it can be converted
to sound by the earphone, which is connected to the detector.
The rectified current from the detector has
radio frequency
pulses from the carrier frequency in it, which are blocked by the high
inductive reactance and do not pass well through the coils of early date
earphones. Hence, a small
capacitor called a
bypass capacitor
is often placed across the earphone terminals; its low reactance at
radio frequency bypasses these pulses around the earphone to ground. In some sets the earphone cord had enough capacitance that this component could be omitted.
In a cat's whisker detector only certain sites on the crystal surface
functioned as rectifying junctions, and the device was very sensitive
to the pressure of the crystal-wire contact, which could be disrupted by
the slightest vibration.
Therefore, a usable contact point had to be found by trial and error
before each use. The operator dragged the wire across the crystal
surface until a radio station or "static" sounds were heard in the
earphones.
Alternatively, some radios
(circuit, right) used a battery-powered
buzzer attached to the input circuit to adjust the detector.
The spark at the buzzer's electrical contacts served as a weak source
of static, so when the detector began working, the buzzing could be
heard in the earphones. The buzzer was then turned off, and the radio
tuned to the desired station.
Galena (lead sulfide) was probably the most common crystal used in cat's whisker detectors, but various other types of crystals were also used, the most common being
iron pyrite (fool's gold, FeS
2),
silicon,
molybdenite (MoS
2),
silicon carbide (carborundum, SiC), and a
zincite-
bornite (ZnO-Cu
5FeS
4) crystal-to-crystal junction trade-named
Perikon.
Crystal radios have also been improvised from a variety of common objects, such as blue steel
razor blades and
lead pencils, rusty needles, and pennies In these, a
semiconducting layer of oxide or sulfide on the metal surface is usually responsible for the rectifying action.
In modern sets, a
semiconductor diode is used for the detector, which is much more reliable than a cat's whisker detector and requires no adjustments.
Germanium diodes (or sometimes
Schottky diodes) are used instead of
silicon diodes, because their lower forward voltage drop (roughly 0.3V compared to 0.6V
) makes them more sensitive.
All semiconductor detectors function rather inefficiently in crystal
receivers, because the low voltage input to the detector is too low to
result in much difference between forward better conduction direction,
and the reverse weaker conduction. To improve the sensitivity of some of
the early crystal detectors, such as silicon carbide, a small
forward bias voltage was applied across the detector by a battery and
potentiometer.
The bias moves the diode's operating point higher on the detection
curve producing more signal voltage at the expense of less signal
current (higher impedance). There is a limit to the benefit that this
produces, depending on the other impedances of the radio. This improved
sensitivity was caused by moving the DC operating point to a more
desirable voltage-current operating point (impedance) on the junction's
I-V curve. The battery did not power the radio, but only provided the biasing voltage which required little power.
Earphones
The requirements for earphones used in crystal sets are different
from earphones used with modern audio equipment. They have to be
efficient at converting the electrical signal energy to sound waves,
while most modern earphones sacrifice efficiency in order to gain
high fidelity reproduction of the sound. In early homebuilt sets, the earphones were the most costly component.
The early earphones used with wireless-era crystal sets had
moving iron drivers that worked in a way similar to the horn
loudspeakers of the period; modern loudspeakers use a moving-coil principle. Each earpiece contained a permanent
magnet about which was a coil of wire which formed a second
electromagnet. Both magnetic poles were close to a steel diaphram of the speaker. When the
audio signal from the radio was passed through the electromagnet's windings, current was caused to flow in the coil which created a varying
magnetic field
that augmented or diminished that due to the permanent magnet. This
varied the force of attraction on the diaphragm, causing it to vibrate.
The vibrations of the diaphragm push and pull on the air in front of it,
creating sound waves. Standard headphones used in telephone work had a
low
impedance,
often 75 Ω, and required more current than a crystal radio could
supply. Therefore, the type used with crystal set radios (and other
sensitive equipment) was wound with more turns of finer wire giving it a
high impedance of 2000-8000 Ω.
Modern crystal sets use
piezoelectric crystal earpieces, which are much more sensitive and also smaller.
They consist of a
piezoelectric
crystal with electrodes attached to each side, glued to a light
diaphragm. When the audio signal from the radio set is applied to the
electrodes, it causes the crystal to vibrate, vibrating the diaphragm.
Crystal earphones are designed as
ear buds
that plug directly into the ear canal of the wearer, coupling the sound
more efficiently to the eardrum. Their resistance is much higher
(typically megohms) so they do not greatly "load" the tuned circuit,
allowing increased
selectivity of the receiver. The piezoelectric earphone's higher resistance, in parallel with its capacitance of around 9 pF, creates a
filter that allows the passage of low frequencies, but blocks the higher frequencies.
In that case a bypass capacitor is not needed (although in practice a
small one of around 0.68 to 1 nF is often used to help improve quality),
but instead a 10-100 kΩ resistor must be added in parallel with the
earphone's input.
Although the low power produced by crystal radios is typically insufficient to drive a
loudspeaker, some homemade 1960s sets have used one, with an audio
transformer to match the low impedance of the speaker to the circuit.
Similarly, modern low-impedance (8 Ω) earphones cannot be used
unmodified in crystal sets because the receiver does not produce enough
current to drive them. They are sometimes used by adding an audio
transformer to match their impedance with the higher impedance of the
driving antenna circuit.
Use as a power source
A
crystal radio tuned to a strong local transmitter can be used as a
power source for a second amplified receiver of a distant station that
cannot be heard without amplification.
There is a long history of unsuccessful attempts and unverified
claims to recover the power in the carrier of the received signal
itself. Traditional crystal sets use half-wave
rectifiers. As
AM signals have a
modulation factor of only 30% by voltage at peaks
, no more than 9% of received signal power (

)
is actual audio information, and 91% is just rectified DC voltage.
Given that the audio signal is unlikely to be at peak all the time, the
ratio of energy is, in practice, even greater. Considerable effort was
made to convert this DC voltage into sound energy. Some earlier attempts
include a one-
transistor
amplifier in 1966. Sometimes efforts to recover this power are confused
with other efforts to produce a more efficient detection. This history continues now with designs as elaborate as "inverted two-wave switching power unit".
X . IIII
Electro-optical sensor
in detector aplication
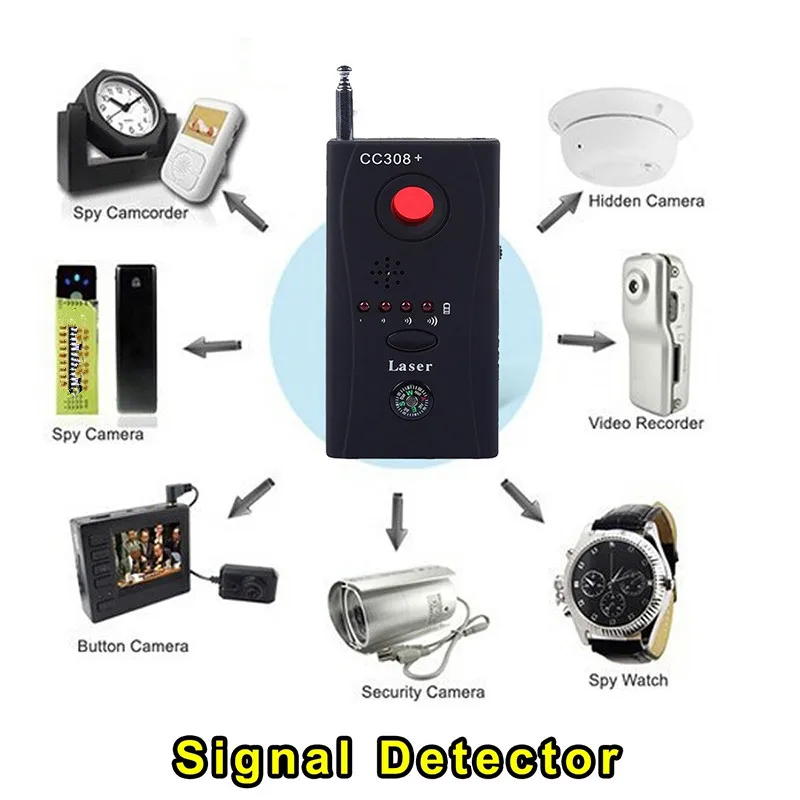
Electro-optical sensors are electronic detectors that convert
light, or a change in light, into an electronic signal. They are used in many industrial and consumer applications, for example:
Function
An
optical sensor converts light rays into electronic signals. It measures
the physical quantity of light and then translates it into a form that
is readable by an instrument. An optical sensor is generally part of a
larger system that integrates a source of light, a measuring device and
the optical sensor. This is often connected to an electrical trigger.
The trigger reacts to a change in the signal within the light sensor. An
optical sensor can measure the changes from one or several light beams.
When a change occurs, the light sensor operates as a photoelectric
trigger and therefore either increases or decreases the electrical
output. An optical switch enables signals in optical fibres or
integrated optical circuits
to be switched selectively from one circuit to another. An optical
switch can operate by mechanical means or by electro-optic effects,
magneto-optic effects as well as by other methods. Optical switches are
optoelectronic devices which can be integrated with integrated or
discrete microelectronic circuits.
Types of Optical Sensors and Switches
There are many different kinds of optical sensors, the most common types are:
- Photoconductive devices convert a change of incident light into a change of resistance.
- Photovoltaics, commonly known as solar cells, convert an amount of incident light into an output voltage.
- Photodiodes convert an amount of incident light into an output current.
- Phototransistors are a type of bipolar transistor
where the base-collector junction is exposed to light. This results in
the same behaviour of a photodiode, but with an internal gain.
Optical Switches are usually used in
optical fibers, where the
electro-optic effect is used to switch one circuit to another. These switches can be implemented with, for example,
microelectromechanical systems or
piezoelectric systems.
Applications
Electro-optical
sensors are used whenever light needs to be converted to energy.
Because of this, electro-optical sensors can be seen almost anywhere.
Common applications are
smartphones where sensors are used to adjust screen brightness, and
smartwatches in which sensors are used to measure the wearer's heartbeat.
Optical sensors can be found in the energy field to monitor
structures that generate, produce, distribute, and convert electrical
power. The distributed and nonconductive nature of optical fibres makes
optical sensors perfect for oil and gas applications, including pipeline
monitoring. They can also be found in wind turbine blade monitoring,
offshore platform monitoring, power line monitoring and downhole
monitoring. Other applications include the civil and transportation
fields such as bridge, airport landing strip, dam, railway, airplane,
wing, fuel tank and ship hull monitoring.
Among other applications, optical switches can be found in thermal
methods which vary the refraction index in one leg of an interferometer
in order to switch the signal, MEMS approaches involving arrays of
micromirrors that can deflect an optical signal to the appropriate
receiver, piezoelectric beam steering liquid crystals which rotate
polarized light depending on the applied electric field and
acousto-optic methods which change the refraction index as a result of
strain induced by an acoustic field to deflect light.
Another important application of optical sensor is to measure the
concentration of different compounds by both visible and
infrared spectroscopy.
Light fixture
A large number of light fixtures and lamps at a store.
A
light fixture (US English),
light fitting (UK English), or
luminaire is an electrical device used to create artificial light by use of an
electric lamp.
All light fixtures have a fixture body and a light socket to hold the
lamp and allow for its replacement. Fixtures may also have a switch to
control the light, either attached to the lamp body or attached to the
power cable. Permanent light fixtures, such as dining room chandeliers,
may use a wall switch to turn them on and off; as such, these fixtures
may have no switch on the fixture itself. Fixtures require an
electrical connection to a power source, typically
AC mains
power, but also battery power for camping or emergency lights.
Permanent lighting fixtures may be directly wired. Moveable lamps have a
plug and cord so that they can be plugged into a wall socket. Light fixtures may also have other features, such as
reflectors for directing the light, an
aperture (with or without a
lens), an outer shell or housing for lamp alignment and protection, an
electrical ballast or
power supply,
and a shade to diffuse the light or direct it towards a workspace
(e.g., a desk lamp). A wide variety of special light fixtures are
created for use in the
automotive lighting industry,
aerospace, marine and
medicine sectors.
Portable light fixtures are often called "lamps", as in
table lamp or
desk lamp. In
technical terminology, the
lamp is the light source, which is typically called the
light bulb. The term
luminaire is recommended by the
International Electrotechnical Commission (IEC) for technical use.
a bright cloud
Fixture manufacturing began soon after production of the incandescent
light bulb. When practical uses of fluorescent lighting were realized
after 1924, the three leading companies to produce various fixtures were
Lightolier,
Artcraft Fluorescent Lighting Corporation, and Globe Lighting in the United States.
Fixture types
Light fixtures are classified by how the fixture is installed, the light function or lamp type.
Free-standing or portable
- Table lamp fixtures, standard lamp fixtures, and office task light luminaires.
- Floor Lamp
- Torch lamp or torchières are floor lamps with an upward facing shade. They provide general lighting to the rest of the room.
- Gooseneck lamp
- Bouillotte lamp: see Bouillotte
Fixed
- Ceiling Dome — Also called the light source(s) are hidden behind a
translucent dome typically made of glass, with some combination of
frosting and surface texturing to diffuse the light. These can be flush
mount fixtures which are mounted right up against the ceiling, or
semi-flush fixtures which are separated by a small distance (usually
about 3-12").
- Open ceiling dome — the translucent dome is suspended a short
distance below the ceiling by a mechanism that is hidden with the
exception of a screw-knob or other device appearing on the outer dome
face, and pulling this knob releases the dome
- Enclosed ceiling dome The translucent dome mates with a ring that is mounted flush with the ceiling
- Recessed light
— the protective housing is concealed behind a ceiling or wall, leaving
only the fixture itself exposed. The ceiling-mounted version is often
called a downlight.
- "Cans" with a variety of lamps — this term is jargon for inexpensive downlighting
products that are recessed into the ceiling, or sometimes for uplights
placed on the floor. The name comes from the shape of the housing. The
term "pot lights" is often used in Canada and parts of the US.
- Cove light — recessed into the ceiling in a long box against a wall.
- Troffer — recessed fluorescent light fixtures, usually rectangular in shape to fit into a drop ceiling grid.
- Surface-mounted light — the finished housing is exposed, not flush with surface
- Chandelier
- Pendant light — suspended from the ceiling with a chain or pipe
- Sconce — provide up or down lights; can be used to illuminate artwork, architectural details; commonly used in hallways or as an alternative to overhead lighting.
- Track lighting fixture — individual fixtures ("track heads") can be positioned anywhere along the track, which provides electric power.
- Under-cabinet light — mounted below kitchen wall cabinets
- Display Case or Showcase light — shows merchandise on display within
an enclosed case such as jewelry, grocery stores, and chain stores.
- Ceiling fan - May sometimes have a light, often referred to as a light kit mounted to it.
- Emergency lighting or exit sign — connected to a battery backup or to an electric circuit that has emergency power if the mains power fails
- High- and low-bay lighting — typically used for general lighting for industrial buildings and often big-box stores
- Strip lights or Industrial lighting — often long lines of fluorescent lamps used in a warehouse or factory
A garden
solar lamp is an example of landscape lighting
- Outdoor lighting and landscape lighting — used to illuminate walkways, parking lots, roadways, building exteriors and architectural details, gardens, and parks.
- High-mast, usually pole- or stanchion-mounted — for landscape, roadways, and parking lots
- Bollard
— A type of architectural outdoor lighting that is a short, upright
ground-mounted unit typically used to provide cutoff type illumination
for egress lighting, to light walkways, steps, or other pathways.
- Solar lamp
- Street light
- Yard light
Special-purpose lights
Lamp types
Old table lamps at Archaeological Museum, Sri Lanka
- Xenon arc lamp, Yablochkov candle
- Fluorescent lamp, compact fluorescent lamp (CFL), Induction lamp, blacklight.
- Betty lamp, butter lamp, carbide lamp, gas lighting, kerosene lamp, oil lamp, rush light, torch, candle, Limelight, gas mantle
- Safety lamps: Davy lamp & Geordie lamp
- Mercury-vapor lamp, Metal-halide (HMI, HQI, CDM), Sodium vapor or "high-pressure sodium"
- A-lamp, Parabolic aluminized reflector lamp (PAR), reflector lamp (R), bulged reflector lamp (BR) (refer to lamp shapes)
Light-fixture controls
Position sensor :
A position
sensor
is any device that permits position measurement. It can either be an
absolute position sensor or a relative one (displacement sensor).
Position sensors can be linear, angular, or multi-axis.
Some position sensors available today:
Flash (photography)
Video demonstration of high speed flash photography.
A
flash is a device used in
photography producing a flash of
artificial light (typically 1/1000 to 1/200 of a second) at a
color temperature of about 5500 K (
Kelvin)
[citation needed]
to help illuminate a scene. A major purpose of a flash is to illuminate
a dark scene. Other uses are capturing quickly moving objects or
changing the quality of light.
Flash refers either to the flash of light itself or to the
electronic flash unit
discharging the light. Most current flash units are electronic, having
evolved from single-use flashbulbs and flammable powders. Modern
cameras often activate flash units automatically.
Flash units are commonly built directly into a camera. Some cameras
allow separate flash units to be mounted via a standardized "accessory
mount" bracket (a
hot shoe). In professional studio equipment, flashes may be large, standalone units, or
studio strobes, powered by special battery packs or connected to
mains power. They are either synchronized with the camera using a
flash synchronization
cable or radio signal, or are light-triggered, meaning that only one
flash unit needs to be synchronized with the camera, and in turn
triggers the other units, called
slaves.
Types of flash
Flash-lamp
Studies of
magnesium by Bunsen and
Roscoe
in 1859 showed that burning this metal produced a light with similar
qualities to daylight. The potential application to photography inspired
Edward Sonstadt to investigate methods of manufacturing magnesium so
that it would burn reliably for this use. He applied for patents in 1862
and by 1864 had started the Manchester Magnesium Company with Edward
Mellor. With the help of engineer
William Mather,
who was also a director of the company, they produced flat magnesium
ribbon, which was said to burn more consistently and completely so
giving better illumination than round wire. It also had the benefit of
being a simpler and cheaper process than making round wire.
[1] Mather was also credited with the invention of a holder for the ribbon, which formed a lamp to burn it in.
[2] A variety of magnesium ribbon holders were produced by other manufacturers, such as the
Pistol Flashmeter,
which incorporated an inscribed ruler that allowed the photographer to
use the correct length of ribbon for the exposure they needed. The
packaging also implies that the magnesium ribbon was not necessarily
broken off before being ignited.
Vintage AHA smokeless flash powder lamp kit, Germany
An alternative to ribbon was
flash powder, a mixture of magnesium powder and
potassium chlorate, introduced by its German inventors
Adolf Miethe
and Johannes Gaedicke in 1887. A measured amount was put into a pan or
trough and ignited by hand, producing a brief brilliant flash of light,
along with the smoke and noise that might be expected from such an
explosive event. This could be a life-threatening activity, especially
if the flash powder was damp. An electrically triggered flash lamp was invented by
Joshua Lionel Cowen
in 1899. His patent describes a device for igniting photographers’
flash powder by using dry cell batteries to heat a wire fuse. Variations
and alternatives were touted from time to time and a few found a
measure of success in the marketplace, especially for amateur use. In
1905, one French photographer was using intense non-explosive flashes
produced by a special mechanized
carbon arc lamp to photograph subjects in his studio,
but more portable and less expensive devices prevailed. On through the
1920s, flash photography normally meant a professional photographer
sprinkling powder into the trough of a T-shaped flash lamp, holding it
aloft, then triggering a brief and (usually) harmless bit of
pyrotechnics.
Flashbulbs
Flashbulbs have ranged in size from the diminutive AG-1 to the massive No. 75.
The AG-1 flashbulb, introduced in 1958, used wires protruding from its
base as electrical contacts; this eliminated the need for a separate
metal base.
Flashcubes, Magicubes and Flipflash
Flashcube fitted to a Kodak Instamatic camera, showing both unused (left) and used (right) bulbs
Undersides of Flashcube (left) and Magicube (right) cartridges
"Flip flash" type cartridge
Electronic flash
Electronic
flash was developed in the late 1950s while flashbulbs were still in
general use. Early units were expensive and often large and heavy as the
power unit was a separate unit to the flash gun head and was powered by
a large
lead-acid battery,
and usually carried by the photographer by means of a shoulder strap.
Towards the end of the 1960s, the size had dropped to the point where
they were matching the size of conventional bulb flash guns (though the
price, having dropped, was still an obstacle). The electronic flash
system eventually superseded bulb guns as prices came down, rendering
flashbulbs virtually obsolete.
A typical electronic flash unit has
electronic circuitry to charge a high-capacity
capacitor to several hundred
volts.
When the flash is triggered by the shutter's flash synchronization
contact, the capacitor is discharged almost instantaneously through a
flash tube,
producing a flash of very brief duration almost instantaneously (i.e.,
the flash duration, often around 1/1000 of a second, is shorter than
most practical shutter speeds, and full brightness is reached before the
shutter has time to close appreciably).
Synchronization
of full flash brightness with maximum shutter opening was problematic
with bulbs which took an appreciable time to ignite and reach full
brightness; electronic flash does not have these difficulties.
Simple electronic flash units are often mounted on or near the camera; many inexpensive
cameras have an electronic flash unit built in.
Two professional xenon tube flashes
Some lenses have built-in (ring-)flash lights for shadow free macro photography, but there are also accessory ring flashes available.
In a photographic studio, more powerful and flexible studio flash systems are used. They usually contain a
modeling light, an
incandescent light bulb
close to the flash tube; the continuous illumination of the modeling
light lets the photographer visualize the effect of the flash. A system
may comprise multiple synchronised flashes for multi-source lighting.
The strength of a flash device is often indicated in terms of a
guide number designed to simplify exposure setting. The energy released by larger studio flash units, such as
monolights, is indicated in
watt-seconds.
The Nikon brand name for its electronic flash units,
Speedlight, is frequently used as a generic term for electronic flash equipment.
High speed flash
An
air-gap flash is a high-voltage device that discharges a flash of light with an exceptionally short duration, often much less than one
microsecond.
These are commonly used by scientists or engineers for examining
extremely fast-moving objects or reactions, famous for producing images
of
bullets tearing through light bulbs and balloons (see
Harold Eugene Edgerton). An example of a process by which to create a high speed flash is the
exploding wire method.
A photo of a
Smith & Wesson Model 686 firing, taken with a high speed
air-gap flash.
The photo was taken in a darkened room, with camera's shutter open and
the flash was triggered by the sound of the shot using a microphone.
Multi-flash
A
camera that implements multiple flashes can be used to find depth edges
or create stylized images. Such a camera has been developed by
researchers at the
Mitsubishi Electric Research Laboratories
(MERL). Successive flashing of strategically placed flash mechanisms
results in shadows along the depths of the scene. This information can
be manipulated to suppress or enhance details or capture the intricate
geometric features of a scene (even those hidden from the eye), to
create a non-photorealistic image form. Such images could be useful in
technical or medical imaging.
Flash intensity
Unlike
flashbulbs, the intensity of an electronic flash can be adjusted on
some units. To do this, smaller flash units typically vary the capacitor
discharge time, whereas larger (e.g., higher power, studio) units
typically vary the capacitor charge. Color temperature can change as a
result of varying the capacitor charge, thus making color corrections
necessary. Due to advances in semiconductor technology, some studio
units can now control intensity by varying the discharge time and
thereby provide consistent color temperature.
Flash intensity is typically measured in stops or in fractions (1,
1/2, 1/4, 1/8 etc.). Some monolights display an "EV Number", so that a
photographer can know the difference in brightness between different
flash units with different watt-second ratings. EV10.0 is defined as
6400 watt-seconds, and EV9.0 is one stop lower, i.e. 3200 watt-seconds.
Flash duration
Flash duration is commonly described by two numbers that are expressed in fractions of a second:
- t.1 is the length of time the light intensity is above 0.1 (10%) of the peak intensity
- t.5 is the length of time the light intensity is above 0.5 (50%) of the peak intensity
For example, a single flash event might have a t.5 value of 1/1200
and t.1 of 1/450. These values determine the ability of a flash to
"freeze" moving subjects in applications such as sports photography.
In cases where intensity is controlled by capacitor discharge time,
t.5 and t.1 decrease with decreasing intensity. Conversely, in cases
where intensity is controlled by capacitor charge, t.5 and t.1 increase
with decreasing intensity due to the non-linearity of the capacitor's
discharge curve.
Flash LED used in phones
High-current flash
LEDs
are used as flash sources in camera phones, although they are not yet
at the power levels to equal xenon flash devices (that are rarely used
in phones) in still cameras. The major advantages of LEDs over xenon
include low voltage operation, higher efficiency, and extreme
miniaturization. The LED flash can also be used for illumination of
video recordings or as an
autofocus assist lamp in low-light conditions.
Focal-plane-shutter synchronization
Electronic flash units have compatibility issues with
focal-plane shutters.
Focal-plane shutters expose using two curtains that cross the sensor.
The first one opens and the second curtain follows it after a delay
equal to the nominal shutter speed. A typical modern focal-plane shutter
takes about 1/200 s to cross the sensor, so at exposure times shorter
than this only part of the sensor is uncovered at any one time.
Electronic flash can have durations as short as 50 µs, so at such short
exposure times only part of the sensor is exposed. This limits the
shutter speed to about 1/200 s when using flash. In the past,
slow-burning single-use flash bulbs allowed the use of focal-plane
shutters at maximum speed because they produced continuous light for the
time taken for the exposing slit to cross the film gate. If these are
found they cannot be used on modern cameras because the bulb must be
fired *before* the first shutter curtain begins to move (M-sync); the
X-sync used for electronic flash normally fires only when the first
shutter curtain reaches the end of its travel.
High-end flash units address this problem by offering a mode, typically called
FP sync or HSS (
High Speed Sync),
which fires the flash tube multiple times during the time the slit
traverses the sensor. Such units require communication with the camera
and are thus dedicated to a particular camera make. The multiple flashes
result in a significant decrease in guide number, since each is only a
part of the total flash power, but it's all that illuminates any
particular part of the sensor. In general, if
s is the shutter speed, and
t is the shutter traverse time, the guide number reduces by
√s / t.
For example, if the guide number is 100, and the shutter traverse time
is 5 ms (a shutter speed of 1/200s), and the shutter speed is set to
1/2000 s (0.5 ms), the guide number reduces by a factor of
√0.5 / 5, or about 3.16, so the resultant guide number at this speed would be about 32.
Current (2010) flash units frequently have much lower guide numbers
in HSS mode than in normal modes, even at speeds below the shutter
traverse time. For example, the
Mecablitz 58 AF-1 digital flash unit has a guide number of 58 in normal operation, but only 20 in HSS mode, even at low speeds.
Technique
Image exposed without additional lighting (left) and with fill flash (right)
Lighting produced by direct flash (left) and bounced flash (right)
As well as dedicated studio use, flash may be used as the main light
source where ambient light is inadequate, or as a supplementary source
in more complex lighting situations. Basic flash lighting produces a
hard, frontal light unless modified in some way. Several techniques are used to soften light from the flash or provide other effects.
- Softboxes, diffusers that cover the flash lamp, scatter direct light and reduce its harshness.
- Reflectors, including umbrellas, flat-white backgrounds, drapes and reflector cards are commonly used for this purpose (even with small hand-held flash units).
- Bounce flash is a related technique in which flash is directed onto a reflective surface, for example a white ceiling or a flash umbrella,
which then reflects light onto the subject. It can be used as
fill-flash or, if used indoors, as ambient lighting for the whole scene.
Bouncing creates softer, less artificial-looking illumination than
direct flash, often reducing overall contrast and expanding shadow and
highlight detail, and typically requires more flash power than direct
lighting.
Part of the bounced light can be also aimed directly on the subject by
"bounce cards" attached to the flash unit which increase the efficiency
of the flash and illuminate shadows cast by light coming from the
ceiling. It's also possible to use one's own palm for that purpose,
resulting in warmer tones on the picture, as well as eliminating the
need to carry additional accessories.
- Fill flash or "fill-in flash" describes flash used to supplement ambient light
in order to illuminate a subject close to the camera that would
otherwise be in shade relative to the rest of the scene. The flash unit
is set to expose the subject correctly at a given aperture, while
shutter speed is calculated to correctly expose for the background or
ambient light at that aperture setting. Secondary or slave flash
units may be synchronized to the master unit to provide light from
additional directions. The slave units are electrically triggered by the
light from the master flash. Many small flashes and studio monolights
have optical slaves built in. Wireless radio transmitters, such as PocketWizards, allow the receiver unit to be around a corner, or at a distance too far to trigger using an optical sync.
- Strobe: Some high end units can be set to flash a specified number
of times at a specified frequency. This allows action to be frozen
multiple times in a single exposure.
- Colored gels can also be used to change the color of the flash. Correction gels are commonly used, so that the light of the flash is the same as tungsten lights (using a CTO gel) or fluorescent lights.
- Open flash, Free flash or manually-triggred flash
refers to modes in which the photographer manually triggers the flash
unit to fire independently of the shutter.
Drawbacks
Left:
the distance limitation as seen when taking picture of the wooden
floor. Right: the same picture taken with incandescent ambient light,
using a longer exposure and a higher ISO speed setting. The distance is
no longer restricted, but the colors are unnatural because of a lack of
color temperature compensation, and the picture may suffer from more
grain or noise.
Using a flash in a
museum is mostly prohibited.
- Using on-camera flash will give a very harsh light, which results in
a loss of shadows in the image, because the only lightsource is in
practically the same place as the camera. Balancing the flash power and
ambient lighting or using off-camera flash can help overcome these
issues. Using an umbrella or softbox (the flash will have to be
off-camera for this) makes softer shadows.
- A typical problem with cameras using built-in flash units is the low
intensity of the flash; the level of light produced will often not
suffice for good pictures at distances of over 3 metres (10 ft) or so.
Dark, murky pictures with excessive image noise
or "grain" will result. In order to get good flash pictures with simple
cameras, it is important not to exceed the recommended distance for
flash pictures. Larger flashes, especially studio units and monoblocks,
have sufficient power for larger distances, even through an umbrella,
and can even be used against sunlight at short distances.
- The "red-eye effect" is another problem with on camera and ring flash units. Since the retina of the human eye
reflects red light straight back in the direction it came from,
pictures taken from straight in front of a face often exhibit this
effect. It can be somewhat reduced by using the "red eye reduction"
found on many cameras (a pre-flash that makes the subject's irises
contract). However, very good results can be obtained only with a flash
unit that is separated from the camera, sufficiently far from the optical axis, or by using bounce flash, where the flash head is angled to bounce light off a wall, ceiling or reflector.
- On some cameras the flash exposure measuring logic fires a pre-flash
very quickly before the real flash. In some camera/people combinations
this will lead to shut eyes in every picture taken. The blink response
time seems to be around 1/10 of a second. If the exposure flash is fired
at approximately this interval after the TTL measuring flash, people
will be squinting or have their eyes shut. One solution may be the FEL
(flash exposure lock) offered on some more expensive cameras, which
allows the photographer to fire the measuring flash at some earlier
time, long (many seconds) before taking the real picture. Unfortunately
many camera manufacturers do not make the TTL pre-flash interval
configurable.
- Flash distracts people, limiting the number of pictures that can be taken without irritating them.
- Photographing with flash may not be permitted in some museums even after purchasing a permit for taking pictures.
- Flash equipment may take some time to set up, and like any grip
equipment, may need to be carefully secured, especially if hanging
overhead, so it does not fall on anyone. A small breeze can easily
topple a flash with an umbrella on a lightstand if it is not tied down
or sandbagged. Larger equipment (e.g. monoblocks) will need a supply of AC power.
Photoelectric sensor
A
photoelectric sensor, or photo eye, is an
equipment used to discover the distance, absence, or presence of an
object by using a light transmitter, often
infrared, and a
photoelectric
receiver. They are largely used in industrial manufacturing. There are
three different useful types: opposed (through beam), retro-reflective,
and proximity-sensing (diffused).
Conceptual through-beam system to detect unauthorized access to a secure
door. If the beam is damaged, the detector triggers an alarm .
Types
A self-contained photoelectric sensor contains the
optics, along with the
electronics. It requires only a power source. The sensor performs its own
modulation, demodulation,
amplification,
and output switching. Some self-contained sensors provide such options
as built-in control timers or counters. Because of technological
progress, self-contained photoelectric sensors have become increasingly
smaller.
Remote photoelectric sensors used for
remote sensing
contain only the optical components of a sensor. The circuitry for
power input, amplification, and output switching are located elsewhere,
typically in a control panel. This allows the sensor, itself, to be very
small. Also, the controls for the sensor are more accessible, since
they may be bigger.
When space is restricted or the environment too hostile even for remote sensors,
fiber optics
may be used. Fiber optics are passive mechanical sensing components.
They may be used with either remote or self-contained sensors. They have
no electrical circuitry and no moving parts, and can safely pipe light
into and out of hostile environments.
Sensing modes
A
through beam arrangement consists of a receiver located within the
line-of-sight of the transmitter. In this mode, an object is detected
when the light beam is blocked from getting to the receiver from the
transmitter.
A retroreflective arrangement places the transmitter and receiver at
the same location and uses a reflector to bounce the light beam back
from the transmitter to the receiver. An object is sensed when the beam
is interrupted and fails to reach the receiver.
A proximity-sensing (diffused) arrangement is one in which the
transmitted radiation must reflect off the object in order to reach the
receiver. In this mode, an object is detected when the receiver sees the
transmitted source rather than when it fails to see it. As in
retro-reflective sensors, diffuse sensor emitters and receivers are
located in the same housing. But the target acts as the reflector, so
that detection of light is reflected off the disturbance object. The
emitter sends out a beam of light (most often a pulsed infrared, visible
red, or laser) that diffuses in all directions, filling a detection
area. The target then enters the area and deflects part of the beam back
to the receiver. Detection occurs and output is turned on or off when
sufficient light falls on the receiver.
Some photo eyes have two different operational types, light operate
and dark operate. Light operate photo eyes become operational when the
receiver "receives" the transmitter signal. Dark operate photo eyes
become operational when the receiver "does not receive" the transmitter
signal.
The detecting range of a photoelectric sensor is its "field of view",
or the maximum distance from which the sensor can retrieve information,
minus the minimum distance. A minimum detectable object is the smallest
object the sensor can detect. More accurate sensors can often have
minimum detectable objects of minuscule size.
Certain types of
smoke detector use a photoelectric sensor to warn of smoldering fires.
Difference Between Modes
Name |
Advantages |
Disadvantages |
Through-Beam |
- Most accurate
- Longest sensing range
- Very reliable
|
- Must install at two points on system: emitter and receiver
- Costly - must purchase both emitter and receiver
|
Reflective |
- Only slightly less accurate than through-beam
- Sensing range better than diffuse
- Very reliable
|
- Must install at two points on system: sensor and reflector
- Slightly more costly than diffuse
- Sensing range less than through-beam
|
Diffuse |
- Only install at one point
- Cost less than through-beam or reflective
|
- Less accurate than through- beam or reflective
- More setup time involved
|
List of sensors
Acoustic, sound, vibration
Automotive, transportation
Chemical
Electric current, electric potential, magnetic, radio
Environment, weather, moisture, humidity
Flow, fluid velocity
Ionizing radiation, subatomic particles
Navigation instruments
Position, angle, displacement, distance, speed, acceleration
Optical, light, imaging, photon
Pressure
Force, density, level
Thermal, heat, temperature
Proximity, presence
Sensor technology
Other sensors and sensor related properties and concepts
- Actigraphy
- Air pollution sensor
- Analog image processing
- Atomic force microscopy
- Atomic Gravitational Wave Interferometric Sensor
- Attitude control (spacecraft): Horizon sensor, Earth sensor, Sun sensor
- Catadioptric sensor
- Chemoreceptor
- Compressive sensing
- Cryogenic particle detectors
- Dew warning
- Diffusion tensor imaging
- Digital holography
- Electronic tongue
- Fine Guidance Sensor
- Flat panel detector
- Functional magnetic resonance imaging
- Glass break detector
- Heartbeat sensor
- Hyperspectral sensors
- IRIS (Biosensor), Interferometric Reflectance Imaging Sensor
- Laser beam profiler
- Littoral Airborne Sensor/Hyperspectral
- LORROS
- Millimeter wave scanner
- Magnetic resonance imaging
- Moire deflectometry
- Molecular sensor
- Nanosensor
- Nano-tetherball Sensor
- Omnidirectional camera
- Organoleptic sensors
- Optical coherence tomography
- Phase unwrapping techniques
- Polygraph Truth Detection
- Positron emission tomography
- Push broom scanner
- Quantization (signal processing)
- Range imaging
- Scanning SQUID microscope
- Single-Photon Emission Computed Tomography (SPECT)
- Smartdust
- SQUID, Superconducting quantum interference device
- SSIES, Special Sensors-Ions, Electrons, and Scintillation thermal plasma analysis package
- SSMIS, Special Sensor Microwave Imager / Sounder
- Structured-light 3D scanner
- Sun sensor, Attitude control (spacecraft)
- Superconducting nanowire single-photon detector
- Thin-film thickness monitor
- Time-of-flight camera
- TriDAR, Triangulation and LIDAR Automated Rendezvous and Docking
- Unattended Ground Sensors
Photonic integrated circuit
A
photonic integrated circuit (
PIC) or
integrated optical circuit is a device that integrates multiple (at least two) photonic functions and as such is similar to an
electronic integrated circuit.
The major difference between the two is that a photonic integrated
circuit provides functions for information signals imposed on
optical wavelengths typically in the
visible spectrum or near
infrared 850 nm-1650 nm.
The most commercially utilized material platform for photonic integrated circuits is
indium phosphide
(InP), which allows for the integration of various optically active and
passive functions on the same chip. Initial examples of photonic
integrated circuits were simple 2 section
distributed Bragg reflector
(DBR) lasers, consisting of two independently controlled device
sections - a gain section and a DBR mirror section. Consequently, all
modern monolithic tunable lasers, widely tunable lasers, externally
modulated lasers and transmitters, integrated receivers, etc. are
examples of photonic integrated circuits. Current state-of-the-art
devices integrate hundreds of functions onto single chip.
Pioneering work in this arena was performed at Bell Laboratories. Most
notable academic centers of excellence of photonic integrated circuits
in InP are the University of California at Santa Barbara, USA, and the
Eindhoven University of Technology in the Netherlands.
A 2005 development showed that silicon can, even though it is an indirect bandgap material, still be used to generate
laser
light via the Raman nonlinearity. Such lasers are not electrically
driven but optically driven and therefore still necessitate a further
optical pump laser source.
Comparison to electronic integration
Unlike electronic integration where
silicon
is the dominant material, system photonic integrated circuits have been
fabricated from a variety of material systems, including electro-optic
crystals such as
lithium niobate, silica on silicon,
Silicon on insulator, various polymers and
semiconductor materials which are used to make
semiconductor lasers such as
GaAs and
InP.
The different material systems are used because they each provide
different advantages and limitations depending on the function to be
integrated. For instance, silica (silicon dioxide) based PICs have very
desirable properties for passive photonic circuits such as AWGs (see
below) due to their comparatively low losses and low thermal
sensitivity, GaAs or InP based PICs allow the direct integration of
light sources and Silicon PICs enable co-integration of the photonics
with transistor based electronics.
The fabrication techniques are similar to those used in electronic integrated circuits in which
photolithography is used to pattern wafers for etching and material deposition. Unlike electronics where the primary device is the
transistor, there is no single dominant device. The range of devices required on a chip includes low loss interconnect
waveguides, power splitters,
optical amplifiers,
optical modulators, filters,
lasers
and detectors. These devices require a variety of different materials
and fabrication techniques making it difficult to realize all of them on
a single chip.
Newer techniques using resonant photonic interferometry is making way
for UV LEDs to be used for optical computing requirements with much
cheaper costs leading the way to petahertz
PHz consumer electronics.
Examples of photonic integrated circuits
The primary application for photonic integrated circuits is in the area of
fiber-optic communication though applications in other fields such as
biomedical and
photonic computing are also possible.
The
arrayed waveguide grating (AWG) which are commonly used as optical (de)multiplexers in
wavelength division multiplexed (WDM)
fiber-optic communication
systems are an example of a photonic integrated circuit which has
replaced previous multiplexing schemes which utilized multiple discrete
filter elements. Since separating optical modes is a need for
quantum computing, this technology may be helpful to miniaturize quantum computers (see
linear optical quantum computing).
Another example of a photonic integrated chip in wide use today in
fiber-optic communication systems is the externally modulated laser (EML) which combines a
distributed feed back laser diode with an
electro-absorption modulator on a single
InP based chip.
Current status
Photonic integration is currently an active topic in U.S. Defense contracts. It is included by the
Optical Internetworking Forum for inclusion in 100 gigahertz optical networking standards.
Optical computing

Optical or
photonic computing uses
photons produced by
lasers or
diodes for computation. For decades, photons have promised to allow a higher
bandwidth than the
electrons used in conventional computers.
Most research projects focus on replacing current computer components with optical equivalents, resulting in an optical
digital computer system processing
binary data.
This approach appears to offer the best short-term prospects for
commercial optical computing, since optical components could be
integrated into traditional computers to produce an optical-electronic
hybrid. However,
optoelectronic
devices lose 30% of their energy converting electronic energy into
photons and back; this conversion also slows the transmission of
messages. All-optical computers eliminate the need for
optical-electrical-optical (OEO) conversions, thus lessening the need
for electrical power.
Application-specific devices, such as
Synthetic aperture radar and
optical correlators,
have been designed to use the principles of optical computing.
Correlators can be used, for example, to detect and track objects, and to classify serial time-domain optical data.
Optical components for binary digital computer
The fundamental building block of modern electronic computers is the
transistor. To replace electronic components with optical ones, an equivalent
optical transistor is required. This is achieved using materials with a
non-linear refractive index. In particular, materials exist
where the intensity of incoming light affects the intensity of the
light transmitted through the material in a similar manner to the
current response of a bipolar transistor. Such an 'optical transistor'
can be used to create optical
logic gates, which in turn are assembled into the higher level components of the computer's
CPU. These will be nonlinear optical crystals used to manipulate light beams into controlling other light beams.
Controversy
There
are disagreements between researchers about the future capabilities of
optical computers: will they be able to compete with semiconductor-based
electronic computers on speed, power consumption, cost, and size?
Critics note that
real-world logic systems require "logic-level restoration, cascadability,
fan-out
and input–output isolation", all of which are currently provided by
electronic transistors at low cost, low power, and high speed. For
optical logic to be competitive beyond a few niche applications, major
breakthroughs in non-linear optical device technology would be required,
or perhaps a change in the nature of computing itself.
Misconceptions, challenges, and prospects
A significant challenge to optical computing is that computation is a
nonlinear process in which multiple signals must interact. Light, which is an
electromagnetic wave, can only interact with another electromagnetic wave in the presence of electrons in a material,
and the strength of this interaction is much weaker for electromagnetic
waves, such as light, than for the electronic signals in a conventional
computer. This may result in the processing elements for an optical
computer requiring more power and larger dimensions than those for a
conventional electronic computer using transistors.
A further misconception is that since light can travel much faster than the
drift velocity of electrons, and at frequencies measured in
THz, optical transistors should be capable of extremely high frequencies. However, any electromagnetic wave must obey the
transform limit, and therefore the rate at which an optical transistor can respond to a signal is still limited by its
spectral bandwidth. However, in
fiber optic communications, practical limits such as
dispersion often constrain
channels
to bandwidths of 10s of GHz, only slightly better than many silicon
transistors. Obtaining dramatically faster operation than electronic
transistors would therefore require practical methods of transmitting
ultrashort pulses down highly dispersive waveguides.
Photonic logic
Realization of a Photonic Controlled-NOT Gate for use in Quantum Computing
Photonic logic is the use of photons (
light) in
logic gates (NOT, AND, OR, NAND, NOR, XOR, XNOR). Switching is obtained using
nonlinear optical effects when two or more signals are combined.
Resonators are especially useful in photonic logic, since they allow a build-up of energy from
constructive interference, thus enhancing optical nonlinear effects.
Other approaches currently being investigated include photonic logic at a
molecular level, using
photoluminescent chemicals. In a recent demonstration, Witlicki et al. performed logical operations using molecules and
SERS.
Optical fiber
A bundle of optical fibers
Fiber crew installing a 432-count fiber cable underneath the streets of Midtown Manhattan, New York City
A
TOSLINK fiber optic audio cable with red light being shone in one end transmits the light to the other end
An
optical fiber or
optical fibre is a flexible, transparent fiber made by
drawing glass (
silica) or plastic to a diameter slightly thicker than that of a
human hair. Optical fibers are used most often as a means to transmit light between the two ends of the fiber and find wide usage in
fiber-optic communications, where they permit transmission over longer distances and at higher
bandwidths (data rates) than wire cables. Fibers are used instead of
metal wires because signals travel along them with lesser amounts of
loss; in addition, fibers are also immune to
electromagnetic interference, a problem from which metal wires suffer excessively. Fibers are also used for
illumination,
and are wrapped in bundles so that they may be used to carry images,
thus allowing viewing in confined spaces, as in the case of a
fiberscope. Specially designed fibers are also used for a variety of other applications, some of them being
fiber optic sensors and
fiber lasers.
Optical fibers typically include a
transparent core surrounded by a transparent
cladding material with a lower
index of refraction. Light is kept in the core by the phenomenon of
total internal reflection which causes the fiber to act as a
waveguide. Fibers that support many propagation paths or
transverse modes are called
multi-mode fibers (MMF), while those that support a single mode are called
single-mode fibers
(SMF). Multi-mode fibers generally have a wider core diameter and are
used for short-distance communication links and for applications where
high power must be transmitted.
Single-mode fibers are used for most communication links longer than 1,000 meters (3,300 ft).
Being able to join optical fibers with low loss is important in fiber optic communication. This is more complex than joining electrical wire or cable and involves careful
cleaving
of the fibers, precise alignment of the fiber cores, and the coupling
of these aligned cores. For applications that demand a permanent
connection a
fusion splice is common. In this technique, an electric arc is used to melt the ends of the fibers together. Another common technique is a
mechanical splice,
where the ends of the fibers are held in contact by mechanical force.
Temporary or semi-permanent connections are made by means of specialized
optical fiber connectors.
The field of applied science and engineering concerned with the design and application of optical fibers is known as
fiber optics. The term was coined by
Indian physicist Narinder Singh Kapany who is widely acknowledged as the father of fiber optics.
a bright cloud
Daniel Colladon first described this “light fountain” or “light pipe” in an 1842 article titled
On the reflections of a ray of light inside a parabolic liquid stream. This particular illustration comes from a later article by Colladon, in 1884.
Record speeds
Achieving a high data rate and covering a long distance
simultaneously is challenging. To express this, sometimes the product of data rate and distance is specified –
(bit/s)×km or the equivalent
bit×km/s, similar to the
bandwidth-distance product.
- 2006 – Nippon Telegraph and Telephone Corporation transferred 14 terabits per second over a single 160 km long optical fiber
- 2009 – Bell Labs in Villarceaux, France transferred 100 Gbit/s over 7000 km fiber
- 2010 – Nippon Telegraph and Telephone Corporation transferred
69.1 Tbit/s over a single 240 km fiber multiplexing 432 channels,
equating to 171 Gbit/s per channel
- 2012 – Nippon Telegraph and Telephone Corporation transferred 1 Petabit per second over 50 kilometers over a single fiber
Uses
Communication
Optical fiber can be used as a medium for telecommunication and
computer networking because it is flexible and can be bundled as
cables.
It is especially advantageous for long-distance communications, because
light propagates through the fiber with little attenuation compared to
electrical cables. This allows long distances to be spanned with few
repeaters.
The per-channel light signals propagating in the fiber have been modulated at rates as high as 111
gigabits per second (Gbit/s) by
NTT, although 10 or 40 Gbit/s is typical in deployed systems. In June 2013, researchers demonstrated transmission of 400 Gbit/s over a single channel using 4-mode
orbital angular momentum multiplexing.
Each fiber can carry many independent channels, each using a different wavelength of light (
wavelength-division multiplexing
(WDM)). The net data rate (data rate without overhead bytes) per fiber
is the per-channel data rate reduced by the FEC overhead, multiplied by
the number of channels (usually up to eighty in commercial
dense WDM systems as of 2008). As of 2011 the record for bandwidth on a single core was 101 Tbit/s (370 channels at 273 Gbit/s each). The record for a multi-core fiber as of January 2013 was 1.05 petabits per second. In 2009, Bell Labs broke the 100 (petabit per second)×kilometer barrier (15.5 Tbit/s over a single 7,000 km fiber).
For short-distance applications, such as a network in an office building (see
FTTO),
fiber-optic cabling can save space in cable ducts. This is because a
single fiber can carry much more data than electrical cables such as
standard
category 5
Ethernet cabling, which typically runs at 100 Mbit/s or 1 Gbit/s
speeds. Fiber is also immune to electrical interference; there is no
cross-talk between signals in different cables, and no pickup of
environmental noise. Non-armored fiber cables do not conduct
electricity, which makes fiber a good solution for protecting
communications equipment in
high voltage environments, such as
power generation facilities, or metal communication structures prone to
lightning strikes. They can also be used in environments where explosive fumes are present, without danger of ignition.
Wiretapping (in this case,
fiber tapping) is more difficult compared to electrical connections, and there are concentric dual-core fibers that are said to be tap-proof.
Fibers are often also used for short-distance connections between devices. For example, most
high-definition televisions offer a digital audio optical connection. This allows the streaming of audio over light, using the
TOSLINK protocol.
The advantages of optical fiber communication with respect to copper wire systems are:
- Broad bandwidth: A single optical fiber can carry over 3,000,000 full-duplex voice calls or 90,000 TV channels.
- Immunity to electromagnetic interference: Light transmission through optical fibers is unaffected by other electromagnetic radiation
nearby. The optical fiber is electrically non-conductive, so it does
not act as an antenna to pick up electromagnetic signals. Information
traveling inside the optical fiber is immune to electromagnetic interference, even electromagnetic pulses generated by nuclear devices.
- Low attenuation loss over long distances: Attenuation loss
can be as low as 0.2 dB/km in optical fiber cables, allowing
transmission over long distances without the need for repeaters.
- Electrical insulator: Optical fibers do not conduct electricity, preventing problems with ground loops and conduction of lightning. Optical fibers can be strung on poles alongside high voltage power cables.
- Material cost and theft prevention: Conventional cable systems use large amounts of copper. Global copper prices experienced a boom in the 2000s, and copper has been a target of metal theft.
- Security of information passed down the cable: Copper can be tapped with very little chance of detection.
Sensors
Fibers have many uses in remote sensing. In some applications, the
sensor is itself an optical fiber. In other cases, fiber is used to
connect a non-fiberoptic sensor to a measurement system. Depending on
the application, fiber may be used because of its small size, or the
fact that no
electrical power is needed at the remote location, or because many sensors can be
multiplexed
along the length of a fiber by using different wavelengths of light for
each sensor, or by sensing the time delay as light passes along the
fiber through each sensor. Time delay can be determined using a device
such as an
optical time-domain reflectometer.
Optical fibers can be used as sensors to measure
strain,
temperature,
pressure and other quantities by modifying a fiber so that the property to measure modulates the
intensity,
phase,
polarization,
wavelength,
or transit time of light in the fiber. Sensors that vary the intensity
of light are the simplest, since only a simple source and detector are
required. A particularly useful feature of such fiber optic sensors is
that they can, if required, provide distributed sensing over distances
of up to one meter. In contrast, highly localized measurements can be
provided by integrating miniaturized sensing elements with the tip of
the fiber.
These can be implemented by various micro- and nanofabrication
technologies, such that they do not exceed the microscopic boundary of
the fiber tip, allowing such applications as insertion into blood
vessels via hypodermic needle.
Extrinsic fiber optic sensors use an
optical fiber cable, normally a multi-mode one, to transmit
modulated
light from either a non-fiber optical sensor—or an electronic sensor
connected to an optical transmitter. A major benefit of extrinsic
sensors is their ability to reach otherwise inaccessible places. An
example is the measurement of temperature inside
aircraft jet engines by using a fiber to transmit
radiation into a radiation
pyrometer outside the engine. Extrinsic sensors can be used in the same way to measure the internal temperature of
electrical transformers, where the extreme
electromagnetic fields
present make other measurement techniques impossible. Extrinsic sensors
measure vibration, rotation, displacement, velocity, acceleration,
torque, and twisting. A solid state version of the gyroscope, using the
interference of light, has been developed. The
fiber optic gyroscope (FOG) has no moving parts, and exploits the
Sagnac effect to detect mechanical rotation.
Common uses for fiber optic sensors includes advanced intrusion
detection security systems. The light is transmitted along a fiber optic
sensor cable placed on a fence, pipeline, or communication cabling, and
the returned signal is monitored and analyzed for disturbances. This
return signal is digitally processed to detect disturbances and trip an
alarm if an intrusion has occurred.
Optical fibers are widely used as components of optical chemical sensors and optical
biosensors.
Power transmission
Optical fiber can be used to transmit power using a
photovoltaic cell to convert the light into electricity.
While this method of power transmission is not as efficient as
conventional ones, it is especially useful in situations where it is
desirable not to have a metallic conductor as in the case of use near
MRI machines, which produce strong magnetic fields.
Other examples are for powering electronics in high-powered antenna
elements and measurement devices used in high-voltage transmission
equipment.
Other uses
A
frisbee illuminated by fiber optics
Light reflected from optical fiber illuminates exhibited model
Optical fibers have a wide number of applications. They are used as
light guides
in medical and other applications where bright light needs to be shone
on a target without a clear line-of-sight path. In some buildings,
optical fibers route sunlight from the roof to other parts of the
building (see
nonimaging optics).
Optical fiber lamps are used for illumination in decorative applications, including
signs,
art, toys and artificial
Christmas trees.
Swarovski
boutiques use optical fibers to illuminate their crystal showcases from
many different angles while only employing one light source. Optical
fiber is an intrinsic part of the light-transmitting concrete building
product,
LiTraCon.
Use of optical fiber in a decorative lamp or nightlight.
Optical fiber is also used in imaging optics. A coherent bundle of
fibers is used, sometimes along with lenses, for a long, thin imaging
device called an
endoscope,
which is used to view objects through a small hole. Medical endoscopes
are used for minimally invasive exploratory or surgical procedures.
Industrial endoscopes (see
fiberscope or
borescope) are used for inspecting anything hard to reach, such as jet engine interiors. Many
microscopes use fiber-optic light sources to provide intense illumination of samples being studied.
In
spectroscopy,
optical fiber bundles transmit light from a spectrometer to a substance
that cannot be placed inside the spectrometer itself, in order to
analyze its composition. A spectrometer analyzes substances by bouncing
light off and through them. By using fibers, a spectrometer can be used
to study objects remotely.
An optical fiber
doped with certain
rare earth elements such as
erbium can be used as the
gain medium of a
laser or
optical amplifier. Rare-earth-doped optical fibers can be used to provide signal
amplification by splicing a short section of doped fiber into a regular (undoped) optical fiber line. The doped fiber is
optically pumped
with a second laser wavelength that is coupled into the line in
addition to the signal wave. Both wavelengths of light are transmitted
through the doped fiber, which transfers energy from the second pump
wavelength to the signal wave. The process that causes the amplification
is
stimulated emission.
Optical fiber is also widely exploited as a nonlinear medium. The
glass medium supports a host of nonlinear optical interactions, and the
long interaction lengths possible in fiber facilitate a variety of
phenomena, which are harnessed for applications and fundamental
investigation.
Conversely, fiber nonlinearity can have deleterious effects on optical
signals, and measures are often required to minimize such unwanted
effects.
Optical fibers doped with a
wavelength shifter collect
scintillation light in
physics experiments.
Fiber optic sights for handguns, rifles, and shotguns use pieces of optical fiber to improve visibility of markings on the sight.
Principle of operation
An overview of the operating principles of the optical fiber
An optical fiber is a cylindrical
dielectric waveguide (
nonconducting waveguide) that transmits light along its axis, by the process of
total internal reflection. The fiber consists of a
core surrounded by a
cladding layer, both of which are made of
dielectric materials. To confine the optical signal in the core, the
refractive index of the core must be greater than that of the cladding. The boundary between the core and cladding may either be abrupt, in
step-index fiber, or gradual, in
graded-index fiber.
Index of refraction
The index of refraction (or refractive index) is a way of measuring the
speed of light in a material. Light travels fastest in a
vacuum,
such as in outer space. The speed of light in a vacuum is about 300,000
kilometers (186,000 miles) per second. The refractive index of a medium
is calculated by dividing the speed of light in a vacuum by the speed
of light in that medium. The refractive index of a vacuum is therefore
1, by definition. A typical singlemode fiber used for telecommunications
has a cladding made of pure silica, with an index of 1.444 at 1,500 nm,
and a core of doped silica with an index around 1.4475.
The larger the index of refraction, the slower light travels in that
medium. From this information, a simple rule of thumb is that a signal
using optical fiber for communication will travel at around 200,000
kilometers per second. To put it another way, the signal will take 5
milliseconds
to travel 1,000 kilometers in fiber. Thus a phone call carried by fiber
between Sydney and New York, a 16,000-kilometer distance, means that
there is a minimum delay of 80 milliseconds (about

of a second) between when one caller speaks and the other hears. (The
fiber in this case will probably travel a longer route, and there will
be additional delays due to communication equipment switching and the
process of encoding and decoding the voice onto the fiber).
Total internal reflection
When light traveling in an optically dense medium hits a boundary at a steep angle (larger than the
critical angle
for the boundary), the light is completely reflected. This is called
total internal reflection. This effect is used in optical fibers to
confine light in the core. Light travels through the fiber core,
bouncing back and forth off the boundary between the core and cladding.
Because the light must strike the boundary with an angle greater than
the critical angle, only light that enters the fiber within a certain
range of angles can travel down the fiber without leaking out. This
range of angles is called the
acceptance cone
of the fiber. The size of this acceptance cone is a function of the
refractive index difference between the fiber's core and cladding.
In simpler terms, there is a maximum angle from the fiber axis at
which light may enter the fiber so that it will propagate, or travel, in
the core of the fiber. The
sine of this maximum angle is the
numerical aperture
(NA) of the fiber. Fiber with a larger NA requires less precision to
splice and work with than fiber with a smaller NA. Single-mode fiber has
a small NA.
Multi-mode fiber
A laser bouncing down an
acrylic rod, illustrating the total internal reflection of light in a multi-mode optical fiber.
Fiber with large core diameter (greater than 10 micrometers) may be analyzed by
geometrical optics. Such fiber is called
multi-mode fiber, from the electromagnetic analysis (see below). In a step-index multi-mode fiber,
rays
of light are guided along the fiber core by total internal reflection.
Rays that meet the core-cladding boundary at a high angle (measured
relative to a line
normal to the boundary), greater than the
critical angle
for this boundary, are completely reflected. The critical angle
(minimum angle for total internal reflection) is determined by the
difference in index of refraction between the core and cladding
materials. Rays that meet the boundary at a low angle are refracted from
the
core into the cladding, and do not convey light and hence information along the fiber. The critical angle determines the
acceptance angle of the fiber, often reported as a
numerical aperture.
A high numerical aperture allows light to propagate down the fiber in
rays both close to the axis and at various angles, allowing efficient
coupling of light into the fiber. However, this high numerical aperture
increases the amount of
dispersion as rays at different angles have different
path lengths and therefore take different times to traverse the fiber.
In graded-index fiber, the index of refraction in the core decreases
continuously between the axis and the cladding. This causes light rays
to bend smoothly as they approach the cladding, rather than reflecting
abruptly from the core-cladding boundary. The resulting curved paths
reduce multi-path dispersion because high angle rays pass more through
the lower-index periphery of the core, rather than the high-index
center. The index profile is chosen to minimize the difference in axial
propagation speeds of the various rays in the fiber. This ideal index
profile is very close to a
parabolic relationship between the index and the distance from the axis.
Single-mode fiber
1. Core: 8 µm diameter
2. Cladding: 125 µm dia.
3. Buffer: 250 µm dia.
4. Jacket: 400 µm dia.
Fiber with a core diameter less than about ten times the
wavelength of the propagating light cannot be modeled using geometric optics. Instead, it must be analyzed as an
electromagnetic structure, by solution of
Maxwell's equations as reduced to the
electromagnetic wave equation. The electromagnetic analysis may also be required to understand behaviors such as speckle that occur when
coherent light propagates in multi-mode fiber. As an optical waveguide, the fiber supports one or more confined
transverse modes by which light can propagate along the fiber. Fiber supporting only one mode is called
single-mode or
mono-mode fiber.
The behavior of larger-core multi-mode fiber can also be modeled using
the wave equation, which shows that such fiber supports more than one
mode of propagation (hence the name). The results of such modeling of
multi-mode fiber approximately agree with the predictions of geometric
optics, if the fiber core is large enough to support more than a few
modes.
The waveguide analysis shows that the light energy in the fiber is
not completely confined in the core. Instead, especially in single-mode
fibers, a significant fraction of the energy in the bound mode travels
in the cladding as an
evanescent wave.
The most common type of single-mode fiber has a core diameter of 8–10 micrometers and is designed for use in the
near infrared.
The mode structure depends on the wavelength of the light used, so that
this fiber actually supports a small number of additional modes at
visible wavelengths. Multi-mode fiber, by comparison, is manufactured
with core diameters as small as 50 micrometers and as large as hundreds
of micrometers. The
normalized frequency V for this fiber should be less than the first zero of the
Bessel function J0 (approximately 2.405).
Special-purpose fiber
Some
special-purpose optical fiber is constructed with a non-cylindrical
core and/or cladding layer, usually with an elliptical or rectangular
cross-section. These include
polarization-maintaining fiber and fiber designed to suppress
whispering gallery mode
propagation. Polarization-maintaining fiber is a unique type of fiber
that is commonly used in fiber optic sensors due to its ability to
maintain the polarization of the light inserted into it.
Photonic-crystal fiber
is made with a regular pattern of index variation (often in the form of
cylindrical holes that run along the length of the fiber). Such fiber
uses
diffraction
effects instead of or in addition to total internal reflection, to
confine light to the fiber's core. The properties of the fiber can be
tailored to a wide variety of applications.
Mechanisms of attenuation
Light attenuation by
ZBLAN and silica fibers
Attenuation in fiber optics, also known as transmission loss, is the
reduction in intensity of the light beam (or signal) as it travels
through the transmission medium. Attenuation coefficients in fiber
optics usually use units of dB/km through the medium due to the
relatively high quality of transparency of modern optical transmission
media. The medium is usually a fiber of silica glass that confines the
incident light beam to the inside. Attenuation is an important factor
limiting the transmission of a digital signal across large distances.
Thus, much research has gone into both limiting the attenuation and
maximizing the amplification of the optical signal. Empirical research
has shown that attenuation in optical fiber is caused primarily by both
scattering and
absorption.
Single-mode optical fibers can be made with extremely low loss.
Corning's SMF-28 fiber, a standard single-mode fiber for
telecommunications wavelengths, has a loss of 0.17 dB/km at 1550 nm.
For example, an 8 km length of SMF-28 transmits nearly 75% of light at
1,550 nm. It has been noted that if ocean water was as clear as fiber,
one could see all the way to the bottom even of the Marianas Trench in
the Pacific Ocean, a depth of 36,000 feet.
Light scattering
The propagation of light through the core of an optical fiber is
based on total internal reflection of the lightwave. Rough and irregular
surfaces, even at the molecular level, can cause light rays to be
reflected in random directions. This is called
diffuse reflection or
scattering, and it is typically characterized by wide variety of reflection angles.
Light scattering depends on the
wavelength
of the light being scattered. Thus, limits to spatial scales of
visibility arise, depending on the frequency of the incident light-wave
and the physical dimension (or spatial scale) of the scattering center,
which is typically in the form of some specific micro-structural
feature. Since
visible light has a wavelength of the order of one
micrometer (one millionth of a meter) scattering centers will have dimensions on a similar spatial scale.
Thus, attenuation results from the
incoherent scattering of light at internal
surfaces and interfaces.
In (poly)crystalline materials such as metals and ceramics, in addition
to pores, most of the internal surfaces or interfaces are in the form
of
grain boundaries
that separate tiny regions of crystalline order. It has recently been
shown that when the size of the scattering center (or grain boundary) is
reduced below the size of the wavelength of the light being scattered,
the scattering no longer occurs to any significant extent. This
phenomenon has given rise to the production of
transparent ceramic materials.
Similarly, the scattering of light in optical quality glass fiber is
caused by molecular level irregularities (compositional fluctuations) in
the glass structure. Indeed, one emerging school of thought is that a
glass is simply the limiting case of a polycrystalline solid. Within
this framework, "domains" exhibiting various degrees of short-range
order become the building blocks of both metals and alloys, as well as
glasses and ceramics. Distributed both between and within these domains
are micro-structural defects that provide the most ideal locations for
light scattering. This same phenomenon is seen as one of the limiting
factors in the transparency of IR missile domes.
At high optical powers, scattering can also be caused by nonlinear optical processes in the fiber.
UV-Vis-IR absorption
In
addition to light scattering, attenuation or signal loss can also occur
due to selective absorption of specific wavelengths, in a manner
similar to that responsible for the appearance of color. Primary
material considerations include both electrons and molecules as follows:
- At the electronic level, it depends on whether the electron orbitals
are spaced (or "quantized") such that they can absorb a quantum of
light (or photon) of a specific wavelength or frequency in the
ultraviolet (UV) or visible ranges. This is what gives rise to color.
- At the atomic or molecular level, it depends on the frequencies of
atomic or molecular vibrations or chemical bonds, how close-packed its
atoms or molecules are, and whether or not the atoms or molecules
exhibit long-range order. These factors will determine the capacity of
the material transmitting longer wavelengths in the infrared (IR), far
IR, radio and microwave ranges.
The design of any optically transparent device requires the selection
of materials based upon knowledge of its properties and limitations.
The
Lattice absorption
characteristics observed at the lower frequency regions (mid IR to
far-infrared wavelength range) define the long-wavelength transparency
limit of the material. They are the result of the interactive
coupling between the motions of thermally induced vibrations of the constituent
atoms
and molecules of the solid lattice and the incident light wave
radiation. Hence, all materials are bounded by limiting regions of
absorption caused by atomic and molecular vibrations (bond-stretching)in
the far-infrared (>10 µm).
Thus, multi-phonon absorption occurs when two or more phonons
simultaneously interact to produce electric dipole moments with which
the incident radiation may couple. These dipoles can absorb energy from
the incident radiation, reaching a maximum coupling with the radiation
when the frequency is equal to the fundamental vibrational mode of the
molecular dipole (e.g. Si-O bond) in the far-infrared, or one of its
harmonics.
The selective absorption of infrared (IR) light by a particular
material occurs because the selected frequency of the light wave matches
the frequency (or an integer multiple of the frequency) at which the
particles of that material vibrate. Since different atoms and molecules
have different natural frequencies of vibration, they will selectively
absorb different frequencies (or portions of the spectrum) of infrared
(IR) light.
Reflection and transmission of light waves occur because the
frequencies of the light waves do not match the natural resonant
frequencies of vibration of the objects. When IR light of these
frequencies strikes an object, the energy is either reflected or
transmitted.
Manufacturing
Materials
Glass optical fibers are almost always made from
silica, but some other materials, such as
fluorozirconate,
fluoroaluminate, and
chalcogenide glasses as well as crystalline materials like
sapphire,
are used for longer-wavelength infrared or other specialized
applications. Silica and fluoride glasses usually have refractive
indices of about 1.5, but some materials such as the
chalcogenides can have indices as high as 3. Typically the index difference between core and cladding is less than one percent.
Plastic optical fibers
(POF) are commonly step-index multi-mode fibers with a core diameter of
0.5 millimeters or larger. POF typically have higher attenuation
coefficients than glass fibers, 1 dB/m or higher, and this high
attenuation limits the range of POF-based systems.
Silica
Silica exhibits fairly good optical transmission over a wide range of wavelengths. In the
near-infrared
(near IR) portion of the spectrum, particularly around 1.5 μm, silica
can have extremely low absorption and scattering losses of the order of
0.2 dB/km. Such remarkably low losses are possible only because
ultra-pure silicon is available, it being essential for manufacturing
integrated circuits and discrete transistors. A high transparency in the
1.4-μm region is achieved by maintaining a low concentration of
hydroxyl groups (OH). Alternatively, a high OH
concentration is better for transmission in the
ultraviolet (UV) region.
Silica can be drawn into fibers at reasonably high temperatures, and has a fairly broad
glass transformation range.
One other advantage is that fusion splicing and cleaving of silica
fibers is relatively effective. Silica fiber also has high mechanical
strength against both pulling and even bending, provided that the fiber
is not too thick and that the surfaces have been well prepared during
processing. Even simple cleaving (breaking) of the ends of the fiber can
provide nicely flat surfaces with acceptable optical quality. Silica is
also relatively
chemically inert. In particular, it is not
hygroscopic (does not absorb water).
Silica glass can be doped with various materials. One purpose of doping is to raise the
refractive index (e.g. with
germanium dioxide (GeO
2) or
aluminium oxide (Al
2O
3)) or to lower it (e.g. with
fluorine or
boron trioxide (B
2O
3)). Doping is also possible with laser-active ions (for example,
rare earth-doped fibers) in order to obtain active fibers to be used, for example, in fiber amplifiers or
laser
applications. Both the fiber core and cladding are typically doped, so
that the entire assembly (core and cladding) is effectively the same
compound (e.g. an
aluminosilicate, germanosilicate, phosphosilicate or
borosilicate glass).
Particularly for active fibers, pure silica is usually not a very
suitable host glass, because it exhibits a low solubility for rare earth
ions. This can lead to quenching effects due to clustering of dopant
ions. Aluminosilicates are much more effective in this respect.
Silica fiber also exhibits a high threshold for optical damage. This
property ensures a low tendency for laser-induced breakdown. This is
important for fiber amplifiers when utilized for the amplification of
short pulses.
Because of these properties silica fibers are the material of choice
in many optical applications, such as communications (except for very
short distances with plastic optical fiber), fiber lasers, fiber
amplifiers, and fiber-optic sensors. Large efforts put forth in the
development of various types of silica fibers have further increased the
performance of such fibers over other materials.
Fluoride glass
Fluoride glass is a class of non-oxide optical quality glasses composed of
fluorides of various
metals. Because of their low
viscosity, it is very difficult to completely avoid
crystallization while processing it through the glass transition (or drawing the fiber from the melt). Thus, although
heavy metal
fluoride glasses (HMFG) exhibit very low optical attenuation, they are
not only difficult to manufacture, but are quite fragile, and have poor
resistance to moisture and other environmental attacks. Their best
attribute is that they lack the absorption band associated with the
hydroxyl (OH) group (3,200–3,600 cm
−1; i.e., 2,777–3,125 nm or 2.78–3.13 μm), which is present in nearly all oxide-based glasses.
An example of a heavy metal fluoride glass is the
ZBLAN glass group, composed of
zirconium,
barium,
lanthanum,
aluminium, and
sodium fluorides. Their main technological application is as
optical waveguides in both planar and fiber form. They are advantageous especially in the
mid-infrared (2,000–5,000 nm) range.
HMFGs were initially slated for optical fiber applications, because
the intrinsic losses of a mid-IR fiber could in principle be lower than
those of silica fibers, which are transparent only up to about 2 μm.
However, such low losses were never realized in practice, and the
fragility and high cost of fluoride fibers made them less than ideal as
primary candidates. Later, the utility of fluoride fibers for various
other applications was discovered. These include mid-
IR spectroscopy,
fiber optic sensors,
thermometry, and
imaging. Also, fluoride fibers can be used for guided lightwave transmission in media such as YAG (
yttrium aluminium garnet)
lasers at 2.9 μm, as required for medical applications (e.g.
ophthalmology and
dentistry).
Phosphate glass
The P4O10 cagelike structure—the basic building block for phosphate glass.
Phosphate glass constitutes a class of optical glasses composed of
metaphosphates of various metals. Instead of the SiO
4 tetrahedra observed in silicate glasses, the building block for this glass former is
phosphorus pentoxide (P
2O
5), which crystallizes in at least four different forms. The most familiar
polymorph (see figure) comprises molecules of P
4O
10.
Phosphate glasses can be advantageous over silica glasses for optical
fibers with a high concentration of doping rare earth ions. A mix of
fluoride glass and phosphate glass is fluorophosphate glass.
Chalcogenide glass
The
chalcogens—the elements in
group 16 of the
periodic table—particularly
sulfur (S),
selenium (Se) and
tellurium (Te)—react with more
electropositive elements, such as
silver, to form
chalcogenides.
These are extremely versatile compounds, in that they can be
crystalline or amorphous, metallic or semiconducting, and conductors of
ions or
electrons.
Glass containing chalcogenides can be used to make fibers for far infrared transmission.
[citation needed]
Process
Preform
Illustration of the modified chemical vapor deposition (inside) process
Standard optical fibers are made by first constructing a
large-diameter "preform" with a carefully controlled refractive index
profile, and then "pulling" the preform to form the long, thin optical
fiber. The preform is commonly made by three
chemical vapor deposition methods:
inside vapor deposition,
outside vapor deposition, and
vapor axial deposition.
With
inside vapor deposition, the preform starts as a hollow
glass tube approximately 40 centimeters (16 in) long, which is placed
horizontally and rotated slowly on a
lathe. Gases such as
silicon tetrachloride (SiCl
4) or
germanium tetrachloride (GeCl
4) are injected with
oxygen
in the end of the tube. The gases are then heated by means of an
external hydrogen burner, bringing the temperature of the gas up to
1,900
K (1,600 °C, 3,000 °F), where the tetrachlorides react with oxygen to produce
silica or
germania
(germanium dioxide) particles. When the reaction conditions are chosen
to allow this reaction to occur in the gas phase throughout the tube
volume, in contrast to earlier techniques where the reaction occurred
only on the glass surface, this technique is called
modified chemical vapor deposition (MCVD).
The oxide particles then agglomerate to form large particle chains,
which subsequently deposit on the walls of the tube as soot. The
deposition is due to the large difference in temperature between the gas
core and the wall causing the gas to push the particles outwards (this
is known as
thermophoresis).
The torch is then traversed up and down the length of the tube to
deposit the material evenly. After the torch has reached the end of the
tube, it is then brought back to the beginning of the tube and the
deposited particles are then melted to form a solid layer. This process
is repeated until a sufficient amount of material has been deposited.
For each layer the composition can be modified by varying the gas
composition, resulting in precise control of the finished fiber's
optical properties.
In outside vapor deposition or vapor axial deposition, the glass is formed by
flame hydrolysis, a reaction in which silicon tetrachloride and germanium tetrachloride are oxidized by reaction with water (H
2O) in an
oxyhydrogen
flame. In outside vapor deposition the glass is deposited onto a solid
rod, which is removed before further processing. In vapor axial
deposition, a short
seed rod is used, and a porous preform, whose
length is not limited by the size of the source rod, is built up on its
end. The porous preform is consolidated into a transparent, solid
preform by heating to about 1,800 K (1,500 °C, 2,800 °F).
Cross-section of a fiber drawn from a D-shaped preform
Typical communications fiber uses a circular preform. For some applications such as
double-clad fibers another form is preferred. In
fiber lasers based on double-clad fiber, an asymmetric shape improves the
filling factor for
laser pumping.
Because of the surface tension, the shape is smoothed during the
drawing process, and the shape of the resulting fiber does not reproduce
the sharp edges of the preform. Nevertheless, careful polishing of the
preform is important, since any defects of the preform surface affect
the optical and mechanical properties of the resulting fiber. In
particular, the preform for the test-fiber shown in the figure was not
polished well, and cracks are seen with the confocal
optical microscope.
Drawing
The preform, however constructed, is placed in a device known as a
drawing tower,
where the preform tip is heated and the optical fiber is pulled out as a
string. By measuring the resultant fiber width, the tension on the
fiber can be controlled to maintain the fiber thickness.
Coatings
The
light is guided down the core of the fiber by an optical cladding with a
lower refractive index that traps light in the core through total
internal reflection.
The cladding is coated by a buffer that protects it from moisture and physical damage.
[65]
The buffer coating is what gets stripped off the fiber for termination
or splicing. These coatings are UV-cured urethane acrylate composite or
polyimide materials applied to the outside of the fiber during the
drawing process. The coatings protect the very delicate strands of glass
fiber—about the size of a human hair—and allow it to survive the rigors
of manufacturing, proof testing, cabling and installation.
Today’s glass optical fiber draw processes employ a dual-layer
coating approach. An inner primary coating is designed to act as a shock
absorber to minimize attenuation caused by microbending. An outer
secondary coating protects the primary coating against mechanical damage
and acts as a barrier to lateral forces, and may be colored to
differentiate strands in bundled cable constructions.
These fiber optic coating layers are applied during the fiber draw,
at speeds approaching 100 kilometers per hour (60 mph). Fiber optic
coatings are applied using one of two methods:
wet-on-dry and
wet-on-wet.
In wet-on-dry, the fiber passes through a primary coating application,
which is then UV cured—then through the secondary coating application,
which is subsequently cured. In wet-on-wet, the fiber passes through
both the primary and secondary coating applications, then goes to UV
curing.
Fiber optic coatings are applied in concentric layers to prevent
damage to the fiber during the drawing application and to maximize fiber
strength and microbend resistance. Unevenly coated fiber will
experience non-uniform forces when the coating expands or contracts, and
is susceptible to greater signal attenuation. Under proper drawing and
coating processes, the coatings are concentric around the fiber,
continuous over the length of the application and have constant
thickness.
Fiber optic coatings protect the glass fibers from scratches that
could lead to strength degradation. The combination of moisture and
scratches accelerates the aging and deterioration of fiber strength.
When fiber is subjected to low stresses over a long period, fiber
fatigue can occur. Over time or in extreme conditions, these factors
combine to cause microscopic flaws in the glass fiber to propagate,
which can ultimately result in fiber failure.
Three key characteristics of fiber optic waveguides can be affected
by environmental conditions: strength, attenuation and resistance to
losses caused by microbending. External
optical fiber cable
jackets and buffer tubes protect glass optical fiber from environmental
conditions that can affect the fiber’s performance and long-term
durability. On the inside, coatings ensure the reliability of the signal
being carried and help minimize attenuation due to microbending.
Practical issues
Cable construction
In practical fibers, the cladding is usually coated with a tough
resin coating and an additional
buffer layer, which may be further surrounded by a
jacket
layer, usually plastic. These layers add strength to the fiber but do
not contribute to its optical wave guide properties. Rigid fiber
assemblies sometimes put light-absorbing ("dark") glass between the
fibers, to prevent light that leaks out of one fiber from entering
another. This reduces
cross-talk between the fibers, or reduces
flare in fiber bundle imaging applications.
Modern cables come in a wide variety of sheathings and armor,
designed for applications such as direct burial in trenches, high
voltage isolation, dual use as power lines,
installation in conduit, lashing to aerial telephone poles, submarine
installation, and insertion in paved streets. Multi-fiber cable usually
uses colored coatings and/or buffers to identify each strand. The cost
of small fiber-count pole-mounted cables has greatly decreased due to
the high demand for
fiber to the home (FTTH) installations in Japan and South Korea.
Fiber cable can be very flexible, but traditional fiber's loss
increases greatly if the fiber is bent with a radius smaller than around
30 mm. This creates a problem when the cable is bent around corners or
wound around a spool, making
FTTX
installations more complicated. "Bendable fibers", targeted towards
easier installation in home environments, have been standardized as
ITU-T G.657. This type of fiber can be bent with a radius as low as
7.5 mm without adverse impact. Even more bendable fibers have been
developed. Bendable fiber may also be resistant to fiber hacking, in which the
signal in a fiber is surreptitiously monitored by bending the fiber and
detecting the leakage.
Another important feature of cable is cable's ability to withstand
horizontally applied force. It is technically called max tensile
strength defining how much force can be applied to the cable during the
installation period.
Some fiber optic cable versions are reinforced with
aramid yarns or glass yarns as intermediary
strength member.
In commercial terms, usage of the glass yarns are more cost effective
while no loss in mechanical durability of the cable. Glass yarns also
protect the cable core against rodents and termites.
Termination and splicing
Optical fibers are connected to terminal equipment by
optical fiber connectors. These connectors are usually of a standard type such as
FC,
SC,
ST,
LC,
MTRJ, or
SMA. Optical fibers may be connected to each other by connectors or by
splicing, that is, joining two fibers together to form a continuous optical waveguide. The generally accepted splicing method is
arc fusion splicing, which melts the fiber ends together with an
electric arc. For quicker fastening jobs, a “mechanical splice” is used.
Fusion splicing is done with a specialized instrument. The fiber ends
are first stripped of their protective polymer coating (as well as the
more sturdy outer jacket, if present). The ends are
cleaved (cut)
with a precision cleaver to make them perpendicular, and are placed
into special holders in the fusion splicer. The splice is usually
inspected via a magnified viewing screen to check the cleaves before and
after the splice. The splicer uses small motors to align the end faces
together, and emits a small spark between
electrodes at the gap to burn off dust and moisture. Then the splicer generates a larger spark that raises the temperature above the
melting point
of the glass, fusing the ends together permanently. The location and
energy of the spark is carefully controlled so that the molten core and
cladding do not mix, and this minimizes optical loss. A splice loss
estimate is measured by the splicer, by directing light through the
cladding on one side and measuring the light leaking from the cladding
on the other side. A splice loss under 0.1 dB is typical. The complexity
of this process makes fiber splicing much more difficult than splicing
copper wire.
Mechanical fiber splices are designed to be quicker and easier to
install, but there is still the need for stripping, careful cleaning and
precision cleaving. The fiber ends are aligned and held together by a
precision-made sleeve, often using a clear
index-matching gel
that enhances the transmission of light across the joint. Such joints
typically have higher optical loss and are less robust than fusion
splices, especially if the gel is used. All splicing techniques involve
installing an enclosure that protects the splice.
Fibers are terminated in connectors that hold the fiber end precisely
and securely. A fiber-optic connector is basically a rigid cylindrical
barrel surrounded by a sleeve that holds the barrel in its mating
socket. The mating mechanism can be
push and click,
turn and latch (
bayonet mount), or
screw-in (
threaded).
The barrel is typically free to move within the sleeve, and may have a
key that prevents the barrel and fiber from rotating as the connectors
are mated.
A typical connector is installed by preparing the fiber end and
inserting it into the rear of the connector body. Quick-set adhesive is
usually used to hold the fiber securely, and a
strain relief
is secured to the rear. Once the adhesive sets, the fiber's end is
polished to a mirror finish. Various polish profiles are used, depending
on the type of fiber and the application. For single-mode fiber, fiber
ends are typically polished with a slight curvature that makes the mated
connectors touch only at their cores. This is called a
physical contact (PC) polish. The curved surface may be polished at an angle, to make an
angled physical contact (APC)
connection. Such connections have higher loss than PC connections, but
greatly reduced back reflection, because light that reflects from the
angled surface leaks out of the fiber core. The resulting signal
strength loss is called
gap loss. APC fiber ends have low back reflection even when disconnected.
In the 1990s, terminating fiber optic cables was labor-intensive. The
number of parts per connector, polishing of the fibers, and the need to
oven-bake the epoxy in each connector made terminating fiber optic
cables difficult. Today, many connectors types are on the market that
offer easier, less labor-intensive ways of terminating cables. Some of
the most popular connectors are pre-polished at the factory, and include
a gel inside the connector. Those two steps help save money on labor,
especially on large projects. A
cleave
is made at a required length, to get as close to the polished piece
already inside the connector. The gel surrounds the point where the two
pieces meet inside the connector for very little light loss.
Long term performance of the gel is a design consideration, so for the
most demanding installations, factory pre-polished pigtails of
sufficient length to reach the first fusion splice enclosure is normally
the safest approach that minimizes on-site labor.
Free-space coupling
It is often necessary to align an optical fiber with another optical fiber, or with an
optoelectronic device such as a
light-emitting diode, a
laser diode, or a
modulator. This can involve either carefully aligning the fiber and placing it in contact with the device, or can use a
lens
to allow coupling over an air gap. Typically the size of the fiber mode
is much larger than the size of the mode in a laser diode or a
silicon optical chip. In this case a tapered or
lensed fiber
is used to match the fiber mode field distribution to that of the other
element. The lens on the end of the fiber can be formed using
polishing, laser cutting or fusion splicing.
In a laboratory environment, a bare fiber end is coupled using a fiber launch system, which uses a
microscope objective lens to focus the light down to a fine point. A precision
translation stage
(micro-positioning table) is used to move the lens, fiber, or device to
allow the coupling efficiency to be optimized. Fibers with a connector
on the end make this process much simpler: the connector is simply
plugged into a pre-aligned fiberoptic collimator, which contains a lens
that is either accurately positioned with respect to the fiber, or is
adjustable. To achieve the best injection efficiency into single-mode
fiber, the direction, position, size and divergence of the beam must all
be optimized. With good beams, 70 to 90% coupling efficiency can be
achieved.
With properly polished single-mode fibers, the emitted beam has an
almost perfect Gaussian shape—even in the far field—if a good lens is
used. The lens needs to be large enough to support the full numerical
aperture of the fiber, and must not introduce
aberrations in the beam.
Aspheric lenses are typically used.
Fiber fuse
At high optical intensities, above 2
megawatts per square centimeter, when a fiber is subjected to a shock or is otherwise suddenly damaged, a
fiber fuse
can occur. The reflection from the damage vaporizes the fiber
immediately before the break, and this new defect remains reflective so
that the damage propagates back toward the transmitter at 1–3 meters per
second (4–11 km/h, 2–8 mph). The
open fiber control system, which ensures
laser eye safety in the event of a broken fiber, can also effectively halt propagation of the fiber fuse.
In situations, such as undersea cables, where high power levels might
be used without the need for open fiber control, a "fiber fuse"
protection device at the transmitter can break the circuit to keep
damage to a minimum.
Chromatic dispersion
The refractive index of fibers varies slightly with the frequency of
light, and light sources are not perfectly monochromatic. Modulation of
the light source to transmit a signal also slightly widens the frequency
band of the transmitted light. This has the effect that, over long
distances and at high modulation speeds, the different frequencies of
light can take different times to arrive at the receiver, ultimately
making the signal impossible to discern, and requiring extra repeaters.
This problem can be overcome in a number of ways, including the use of a
relatively short length of fiber that has the opposite refractive index
gradient.
conclusion about signal tracer :
Signal tracer
A
signal tracer is a piece of
electronic test equipment used to
troubleshoot radio and other
electronic circuitry.
Usually a very simple device, it normally provides an
amplifier, and a
loudspeaker, often battery-powered and packaged into a small, hand-held
test probe. An optional
diode detector is usually also provided, allowing the detection of
amplitude-modulated signals.
The technician injects a test
signal into the
device under test.
Then, by using the signal tracer, the tech can follow the signal
through the various circuits of the radio receiver. So long as the
signal can be heard, the circuitry up to that point is (at least
minimally) functional. If the signal disappears, however, a
fault can be assumed to be present in the stage of the circuit just passed.
The diode detector is only sensitive to amplitude modulation but even
circuits that are normally used for other modulation schemes (such as
FM radios) can be tested by using an AM test signal for testing the
radio frequency
circuits, then switching to an FM test signal (and switching out the
diode detector) for testing the audio circuits of the radio.
More sophisticated signal tracers may display
digital levels using, for example,
LEDs. For long pulse trains, a
cyclic redundancy check may be calculated and displayed, giving the tech insight into the content of circuits that are switching rapidly.
Test probe
A
test probe is a physical device used to connect
electronic test equipment to a
device under test
(DUT). Test probes range from very simple, robust devices to complex
probes that are sophisticated, expensive, and fragile. Specific types
include
test prods,
oscilloscope probes and
current probes. A test probe is often supplied as a
test lead, which includes the probe, cable and terminating connector.
Voltage probes
Voltage
probes are used to measure voltages present on the DUT. To achieve high
accuracy, the test instrument and its probe must not significantly
affect the voltage being measured. This is accomplished by ensuring that
the combination of instrument and probe exhibit a sufficiently high
impedance that will not load the DUT. For AC measurements, the reactive
component of impedance may be more important than the resistive.
Simple test leads
A pair of simple test leads
A typical
voltmeter probe consists of a single wire
test lead
that has on one end a connector that fits the voltmeter and on the
other end a rigid, tubular plastic section that comprises both a handle
and probe body. The handle allows a person to hold and guide the probe
without influencing the measurement (by becoming part of the electric
circuit) or being exposed to dangerous voltages that might cause
electric shock. Within the probe body, the wire is connected to a rigid, pointed metal tip that contacts the DUT. Some probes allow an
alligator clip to be attached to the tip, thus enabling the probe to be attached to the DUT so that it need not be held in place.
Test leads are usually made with finely stranded wire to keep them
flexible, of wire gauges sufficient to conduct a few amperes of
electric current.
The insulation is chosen to be both flexible and have a breakdown
voltage higher than the voltmeter's maximum input voltage. The many fine
strands and the thick insulation make the wire thicker than ordinary
hookup wire.
Two probes are used together to measure voltage, current, and
two-terminal components such as resistors and capacitors. When making DC
measurements it is necessary to know which probe is positive and which
is negative, so by convention the probes are colored red for positive
and black for negative. Depending upon the accuracy required, they can
be used with signal frequencies ranging from DC to a few
kilohertz.
When sensitive measurements must be made (e.g., very low voltages, or
very low or very high resistances) shields, guards, and techniques such
as four-terminal
Kelvin sensing (using separate leads to carry the measuring current and to sense the voltage) are used.
Tweezer probes
Tweezer probes are a pair of simple probes fixed to a
tweezer mechanism, operated with one hand, for measuring voltages or other electronic circuit parameters between closely spaced pins.
Pogo pins
Spring probes (a.k.a. "
pogo pins") are spring-loaded pins used in electrical
test fixtures
to contact test points, component leads, and other conductive features
of the DUT (Device Under Test). These probes are usually press-fit into
probe sockets, to allow their easy replacement on
test fixtures which may remain in service for decades, testing many thousands of DUTs in
automatic test equipment.
Oscilloscope probes
Oscilloscopes
display the instantaneous waveform of varying electrical quantities,
unlike other instruments which give numerical values of relatively
stable quantities.
Scope probes fall into two main categories: passive and active.
Passive scope probes contain no active electronic parts, such as
transistors, so they require no external power.
Because of the high frequencies often involved,
oscilloscopes
do not normally use simple wires ("flying leads") to connect to the
DUT. Flying leads are likely to pick up interference, so they are not
suitable for low-level signals. Furthermore, the inductance of flying
leads make them unsuitable for high frequency signals. Instead, a
specific
scope probe is used, which uses a
coaxial cable
to transmit the signal from the tip of the probe to the oscilloscope.
This cable has two main benefits: it protects the signal from external
electromagnetic interference, improving accuracy for low-level signals;
and it has a lower inductance than flying leads, making the probe more
accurate for high-frequency signals.
Although coaxial cable has lower inductance than flying leads, it has
higher capacitance: a typical 50 ohm cable has about 90 pF per meter.
Consequently, a one-meter high-impedance direct (1×) coaxial probe may
load the circuit with a capacitance of about 110 pF and a resistance of
1 megohm.
Passive probes
A passive oscilloscope probe with a switch in the probe handle that selects 1× or 10× attenuation
To minimize loading, attenuator probes (e.g., 10× probes) are used. A
typical probe uses a 9 megohm series resistor shunted by a low-value
capacitor to make an RC compensated divider with the cable capacitance
and scope input. The RC time constants are adjusted to match. For
example, the 9 megohm series resistor is shunted by a 12.2 pF capacitor
for a time constant of 110 microseconds. The cable capacitance of 90 pF
in parallel with the scope input of 20 pF (total capacitance 110 pF) and
1 megohm also gives a time constant of 110 microseconds. In practice,
there will be an adjustment so the operator can precisely match the low
frequency time constant (called compensating the probe). Matching the
time constants makes the attenuation independent of frequency. At low
frequencies (where the resistance of
R is much less than the reactance of
C),
the circuit looks like a resistive divider; at higher frequencies
(resistance much greater than reactance), the circuit looks like a
capacitive divider.
The result is a frequency compensated probe for modest frequencies
that presents a load of about 10 megohms shunted by 12 pF. Although such
a probe is an improvement, it does not work when the time scale shrinks
to several cable transit times (transit time is typically 5 ns). In
that time frame, the cable looks like its characteristic impedance, and
there will be reflections from the transmission line mismatch at the
scope input and the probe that causes ringing. The modern scope probe uses lossy low capacitance transmission lines
and sophisticated frequency shaping networks to make the 10× probe
perform well at several hundred megahertz. Consequently, there are other
adjustments for completing the compensation.
A directly connected test probe (so called 1× probe) puts the
unwanted lead capacitance across the circuit under test. For a typical
coaxial cable, loading is of the order of 100pF per meter (the length of a typical test lead).
Attenuator probes minimize capacitive loading with an attenuator, but
reduce the magnitude of the signal delivered to the instrument. A 10×
attenuator will reduce the capacitive load by a factor of about 10. The
attenuator must have an accurate ratio over the whole range of
frequencies of interest; the input impedance of the instrument becomes
part of the attenuator. A DC attenuator with resistive divider is
supplemented with capacitors, so that the frequency response is
predictable over the range of interest.
The RC time constant matching method works as long as the transit
time of the shielded cable is much less than the time scale of interest.
That means that the shielded cable can be viewed as a lumped capacitor
rather than an inductor. Transit time on a 1-meter cable is about 5 ns.
Consequently, these probes will work to a few megahertz, but after that
transmission line effects cause trouble.
At high frequencies, the probe impedance will be low.
The most common design inserts a 9 megohm resistor in series with the
probe tip. The signal is then transmitted from the probe head to the
oscilloscope over a special lossy coaxial cable that is designed to
minimize
capacitance and
ringing. The invention of this cable has been traced to John Kobbe, an engineer working for
Tektronix.
The resistor serves to minimize the loading that the cable capacitance
would impose on the DUT. In series with the normal 1 megohm input
impedance of the oscilloscope, the 9 megohm resistor creates a 10×
voltage divider so such probes are normally known as either
low cap(acitance) probes or
10× probes, often printed with the letter
X or
x instead of the multiplication sign, and usually spoken of as "a times-ten probe".
Because the oscilloscope input has some parasitic capacitance in
parallel with the 1 megohm resistance, the 9 megohm resistor must also
be bypassed by a capacitor to prevent it from forming a severe RC
low-pass filter
with the 'scope's parasitic capacitance. The amount of bypass
capacitance must be carefully matched with the input capacitance of the
oscilloscope so that the capacitors also form a 10× voltage divider. In
this way, the probe provides a uniform 10× attenuation from DC (with the
attenuation provided by the resistors) to very high AC frequencies
(with the attenuation provided by the capacitors).
In the past, the bypass capacitor in the probe head was adjustable
(to achieve this 10× attenuation). More modern probe designs use a
laser-trimmed thick-film
electronic circuit in the head that combines the 9 megohm resistor with
a fixed-value bypass capacitor; they then place a small adjustable
capacitor in parallel with the oscilloscope's input capacitance. Either
way, the probe must be adjusted so that it provides uniform attenuation
at all frequencies. This is referred to as
compensating the probe. Compensation is usually accomplished by probing a 1 kHz
square wave
and adjusting the compensating capacitor until the oscilloscope
displays the most square waveshape. Most oscilloscopes have a 1 kHz
calibration source on their front panels since probe compensation must
be done every time a 10:1 probe is attached to an oscilloscope input.
Newer, faster probes have more complex compensation arrangements and may
occasionally require further adjustments.
100× passive probes are also available, as are some designs specialized for use at very high voltages (up to 25 kV).
Passive probes usually connect to the oscilloscope using a
BNC connector.
Most 10× probes are equivalent to a load of about 10-15 pF and
10 megohms on the DUT, with 100× probes loading the circuit less.
Lo Z probes
Z
0 probes are a specialized type of low-capacitance passive probe used in
low-impedance,
very-high-frequency circuits. They are similar in design to 10× passive
probes but at much lower impedance levels. The probe cables usually
have a characteristic impedance of 50 ohms and connect to oscilloscopes
with a matched 50 ohm (rather than a 1 megohm) input impedance).
High-impedance scope probes are designed for the conventional 1 megohm
oscilloscope, but the 1 megohm input impedance is only at low frequency;
the input impedance is not a constant 1 megohm across the probe's
bandwidth but rather decreases with frequency. For example, a Tektronix
P6139A input impedance starts falling above 10 kHz and is about 100 ohms
at 100 MHz. A different probe technique is needed for high frequency signals.
A high frequency oscilloscope presents a matched load (usually 50
ohms) at its input, which minimizes reflections at the scope. Probing
with a matching 50-ohm transmission line would offer high frequency
performance, but it would unduly load most circuits. An attenuator
(resistive divider) can be used to minimize loading. At the tip, these
probes use a 450 ohm (for 10× attenuation) or 950 ohm (for 20×
attenuation) series resistor. Tektronix sells a 10× divider probe with a 9 GHz bandwidth with a 450 ohm series resistor.
These probes are also called resistive divider probes, since a 50 ohm transmission line presents a purely resistive load.
The
Z0 name refers to the
characteristic impedance
of the oscilloscope and cable. The matched impedances provide better
high-frequency performance than an unmatched passive probe can achieve,
but at the expense of the low 500-ohm load offered by the probe tip to
the DUT. Parasitic capacitance at the probe tip is very low so, for very
high-frequency signals, the Z
0 probe can offer
lower loading than any hi-Z probe and even many active probes.
In principle this type of probe can be used at any frequency, but at
DC and lower frequencies circuits often have high impedances that would
be unacceptably loaded by the probe's low 500 or 1000 ohm probe
impedance. Parasitic impedances limit very-high-frequency circuits to
operating at low impedance, so the probe impedance is less of a problem.
Active scope probes
Active scope probes use a high-impedance high-frequency
amplifier
mounted in the probe head, and a screened lead. The purpose of the
amplifier is not gain, but isolation (buffering) between the circuit
under test and the oscilloscope and cable, loading the circuit with only
a low capacitance and high DC resistance, and matching the oscilloscope
input. Active probes are commonly seen by the circuit under test as a
capacitance of 1 picofarad or less in parallel with 1 megohm resistance.
Probes are connected to the oscilloscope with a cable matching the
characteristic impedance of the oscilloscope input. Tube based active
probes were used before the advent of high-frequency
solid-state electronics, using a small
vacuum tube as
cathode follower amplifier.
Active probes have several disadvantages which have kept them from replacing passive probes for all applications:
- They are several times more expensive than passive probes.
- They require power (but this is usually supplied by the oscilloscope).
- Their dynamic range is limited, sometimes as low as 3 to 5 volts,
and they can be damaged by overvoltage, either from the signal or electrostatic discharge.
Many active probes allow the user to introduce an offset voltage to
allow measurement of voltages with excessive DC level. The total dynamic
range is still limited, but the user may be able to adjust its
centerpoint so that voltages in the range of, for example, zero to five
volts may be measured rather than -2.5 to +2.5.
Because of their inherent low voltage rating, there is little need to
provide high-voltage insulation for operator safety. This allows the
heads of active probes to be extremely small, making them very
convenient for use with modern high-density electronic circuits.
Passive probes and a modest active probe design is discussed in an application note by Williams.
The Tektronix P6201 is an early DC to 900 MHz active FET probe.
At extreme high frequencies a modern digital scope requires that the
user solder a preamp to the DUT to get 50GS/s, 20 GHz performance.
Differential probes
Differential probes are optimized for acquiring
differential signals. To maximize the
common-mode rejection ratio
(CMRR), differential probes must provide two signal paths that are as
nearly identical as possible, matched in overall attenuation, frequency
response, and time delay.
In the past, this was done by designing passive probes with two signal paths, requiring a
differential amplifier
stage at or near the oscilloscope. (A very few early probes fitted the
differential amplifier into a rather-bulky probe head using vacuum
tubes.) With advances in solid-state electronics, it has become
practical to put the differential amplifier directly within the probe
head, greatly easing the requirements on the rest of the signal path
(since it now becomes single-ended rather than differential and the need
to match parameters on the signal path is removed). A modern
differential probe usually has two metal extensions which can be
adjusted by the operator to simultaneously touch the appropriate two
points on the DUT. Very high CMRRs are thereby made possible.
Additional probe features
All
scope probes contain some facility for grounding (earthing) the probe
to the circuit's reference voltage. This is usually accomplished by
connecting a very short pigtail wire from the probe head to ground.
Inductance in the ground wire can lead to distortion in the observed
signal, so this wire is kept as short as possible. Some probes use a
small ground foot instead of any wire, allowing the ground link to be as
short as 10 mm.
Most probes allow a variety of "tips" to be installed. A pointed tip
is the most common, but a seizer probe or "test hook" with a hooked tip
that can secure to the test point, is also commonly used. Tips that have
a small plastic insulating foot with indentations into it can make it
easier to probe very-fine-pitch
integrated circuits;
the indentations mate with the pitch of the IC leads, stabilizing the
probe against the shaking of the user's hand and thereby help to
maintain contact on the desired pin. Various styles of feet accommodate
various pitches of the IC leads. Different types of tips can also be
used for probes for other instruments.
Some probes contain a push button. Pressing the button will either
disconnect the signal (and send a ground signal to the 'scope) or cause
the 'scope to identify the trace in some other way. This feature is very
useful when simultaneously using more than one probe as it lets the
user correlate probes and traces on the 'scope screen.
Some probe designs have additional pins surrounding the BNC or use a
more complex connector than a BNC. These extra connections allow the
probe to inform the oscilloscope of its attenuation factor (10×, 100×,
other). The oscilloscope can then adjust its user displays to
automatically take into account the attenuation and other factors caused
by the probe. These extra pins can also be used to supply power to
active probes.
Some ×10 probes have a "×1/×10" switch. The "×1" position bypasses
the attenuator and compensating network, and can be used when working
with very small signals that would be below the scope's sensitivity
limit if attenuated by ×10.
Interchangeability
Because of their standardized design, passive probes (including Z
0
probes) from any manufacturer can usually be used with any oscilloscope
(although specialized features such as the automatic readout adjustment
may not work). Passive probes with voltage dividers may not be
compatible with a particular scope. The compensation adjustment
capacitor only allows for compensation over a small range of
oscilloscope input capacitance values. The probe compensation range must
be compatible with the oscilloscope input capacitance.
On the other hand, active probes are almost always vendor-specific
due to their power requirements, offset voltage controls, etc. Probe
manufacturers sometimes offer external amplifiers or plug-in AC power
adapters that allow their probes to be used with any oscilloscope.
High-voltage probes
High voltage resistor divider probe
A
high voltage probe allows an ordinary voltmeter to measure
voltages that would otherwise be too high to measure or even
destructive. It does this by reducing the input voltage to a safe,
measurable level with a precision
voltage divider circuit within the probe body.
Probes intended for up to 100 kV typically employ a
resistor voltage divider, with an input resistance of hundreds or thousands of
megohms
to minimize circuit loading. High linearity and accuracy is achieved by
using resistors with extremely low voltage coefficients, in matched
sets that maintain a consistent, precise divider ratio across the
probe's operating temperature. Voltmeters have input resistance that
effectively alters the probe's divider ratio, and
parasitic capacitance that combines with the probe's resistance to form an
RC circuit;
these can easily reduce DC and AC accuracy, respectively, if left
uncompensated. To mitigate these effects, voltage divider probes usually
include additional components that improve frequency response and allow
them to be calibrated for different meter loads.
Even higher voltages can be measured with capacitor divider probes,
though the larger physical size and other mechanical features (e.g.,
corona rings) of these devices often preclude their use as handheld probes.
Current probes
A
current probe
generates a voltage proportional to a current in the circuit being
measured; as the proportionality constant is known, instruments that
respond to voltage can be calibrated to indicate current. Current probes
can be used both by measuring instruments and oscilloscopes.
Sampling resistor
The
classic current probe is a low valued resistor (a "sampling resistor"
or "current shunt") inserted in the current's path. The current is
determined by measuring the voltage drop across the resistor and using
Ohm's law. (
Wedlock & Roberge 1969,
p. 152.) The sampling resistance needs to be small enough not to affect
circuit operation significantly, but large enough to provide a good
reading. The method is valid for both AC and DC measurements. A
disadvantage of this method is the need to break the circuit to
introduce the shunt. Another problem is measuring the voltage across the
shunt when common-mode voltages are present; a differential voltage
measurement is needed.
Alternating current probes
Alternating currents are relatively easy to measure as transformers can be used. A
current transformer
is commonly used to measure alternating currents. The current to be
measured is forced through the primary winding (often a single turn) and
the current through the secondary winding is found by measuring the
voltage across a current-sense resistor (or "burden resistor"). The
secondary winding has a burden resistor to set the current scale. The
properties of a transformer offer many advantages. The current
transformer rejects common mode voltages, so an accurate single-ended
voltage measurement can be made on a grounded secondary. The effective
series resistance

of the primary winding is set by the burden resistor on the secondary winding

and the transformer turns ratio

, where:

.
The core of some current transformers is split and hinged; it is
opened and clipped around the wire to be sensed, then closed, making it
unnecessary to free one end of the conductor and thread it through the
core.
Another clip-on design is the
Rogowski coil. It is a magnetically balanced coil that measures current by electronically evaluating the line integral around a current.
High-frequency, small-signal, passive current probes typically have a
frequency range of several kilohertz to over 100 MHz. The Tektronix
P6022 has a range from 935 Hz to 200 MHz. (
Tektronix 1983, p. 435)
Direct-current probes
Transformers cannot be used to probe
direct currents (DC).
Some DC probe designs use the nonlinear properties of a magnetic material to measure DC.
Other current probes use
Hall effect sensors to measure the
magnetic field around a wire produced by an
electric current through the wire without the need to interrupt the circuit to fit the
probe. They are available for both voltmeters and oscilloscopes. Most
current probes are self-contained, drawing power from a battery or the
instrument, but a few require the use of an external amplifier unit.
Hybrid AC/DC current probes
More
advanced current probes combine a Hall effect sensor with a current
transformer. The Hall effect sensor measures the DC and low frequency
components of the signal and the current transformer measures the high
frequency components. These signals are combined in the amplifier
circuit to yield a wide band signal extending from DC to over 50 MHz. (
Wedlock & Roberge 1969, p. 154) The Tektronix A6302 current probe and AM503 amplifier combination is an example of such a system. (
Tektronix 1983, p. 375) (
Tektronix 1998, p. 571)
Near-field probes
Near-field probes allow the measurement of an
electromagnetic field. They are commonly used to measure electrical
noise and other undesirable
electromagnetic radiation from the DUT, although they can also be used to spy on the workings of the DUT without introducing much
loading into the circuitry.
They are commonly connected to
spectrum analyzers.
Temperature probes
Temperature probes are used to make contact measurements of surface temperatures. They employ a temperature sensor such as a
thermistor,
thermocouple, or
RTD,
to produce a voltage that varies with temperature. In the case of
thermistor and RTD probes, the sensor must be electrically stimulated to
produce a voltage, whereas thermocouple probes do not require
stimulation because a thermocouple will independently produce an output
voltage.
Voltmeters can sometimes be used to measure temperature probes, but
this task is usually delegated to specialized instruments that will
stimulate the probe's sensor (if necessary), measure the probe's output
voltage, and convert the voltage to temperature units.
Demodulator probes
To measure or display the modulating waveform of a
modulated high-frequency signal—for example, an
amplitude-modulated radio signal—a probe fitted with a simple
diode demodulator can be used. The probe will output the modulating waveform without the high-frequency
carrier.